Review | Open Access
Breast cancer stem cells origins and the memory stem cells: clinical significance of biomarkers and the active therapeutic approaches
Bai Chen1, Muhammad Waseem Akram2
1Faculty of Health Sciences, University Teknologi Mara, Shah Alam, Selangor Darul Ehsan 40450, Malaysia.
2Faculty of Pharmacy, University Teknologi Mara, Shah Alam, Selangor Darul Ehsan 40450, Malaysia.
Correspondence: Bai Chen (Faculty of Health Sciences, University Teknologi Mara, Shah Alam, Selangor Darul Ehsan 40450, Malaysia; Email: baichenjack@aliyun.com).
Asia-Pacific Journal of Oncology 2024, 5: 76-84. https://doi.org/10.32948/ajo.2024.10.30
Received: 25 Sep 2024 | Accepted: 30 Oct 2024 | Published online: 08 Nov 2024
Key words breast cancer, breast cancer stem cells, drug resistance, biomarkers
Despite significant advancements in BC diagnosis and therapy, current therapeutic approaches are only partially effective for individuals who come with metastatic BC or who have illness recurrence. Deeper understanding of the mechanisms underlying metastatic BC and the creation of more potent treatments are therefore desperately needed. BC is categorized into various subtypes based on distinct gene expression patterns and histological characteristics [7], and ongoing research aims to uncover the genetic mutations that contribute to tumor initiation and metastasis [8]. Nonetheless, BC results from the intricate interaction of environmental factors, including aspects connected to lifestyle, and hereditary ones. These genetic and environmental factors converge to create significant heterogeneity, which is a major driver of tumor variability. This variation occurs within a single tumor as well as between tumors in various people [9]. New studies employing "omics" technologies, such single-cell DNA and RNA sequencing, are illuminating BC heterogeneity by pinpointing particular cell populations correlated with metastasis and treatment resistance. Noteworthy progress in this field has been made through single-cell sequencing studies, which have revealed the dynamic responses to neoadjuvant chemotherapy in TNBC and identified chemoresistance signatures that can forecast the long-term results of patients [10].
Cancer stem cells (CSCs) simultaneously contribute to and result from tumor heterogeneity. They add to this heterogeneity through their high plasticity, giving rise to cells with diverse phenotypic, functional, and metabolic characteristics. At the same time, they react to numerous micro- and macro-environmental signals, which reflect the complexity of the tumor microenvironment (TME) [11]. In order to self-renew, withstand chemotherapy and radiation, and promote the development of distant metastases, CSCs take advantage of their interactions with the (TME) [12]. In particular, the CSC population is constantly influenced and maintained by non-tumor cells in the microenvironment, such as immune cells and niche components [13]. The ability of CSCs to transition between quiescent and proliferative phases, maximizing survival, demonstrates their adaptability. It has been demonstrated that quiescent CSCs can resist severe environments, elude anticancer treatments, and elude immune identification [11]. Quiescent CSCs are seen in BC and other cancers prior to treatment, build up following radio-chemotherapy, circulate in the bloodstream as circulating tumor cells (CTCs), and can remain in premetastatic areas as disseminated tumor cells (DTCs) for up to 20 years. A characteristic of CSCs is their capacity to dormancy, which is mediated by molecular pathways that are yet poorly understood. Understanding BC dormancy is essential for improving treatment outcomes and avoiding late-stage metastatic relapses, especially in BC that is estrogen receptor (ER)-positive [14]. We provide a review about the origin and microenvironments of BCSC in this review. Lastly, we go over the clinical significance of BCSC and several treatment approaches meant to increase BC patients' metastasis-free survival.
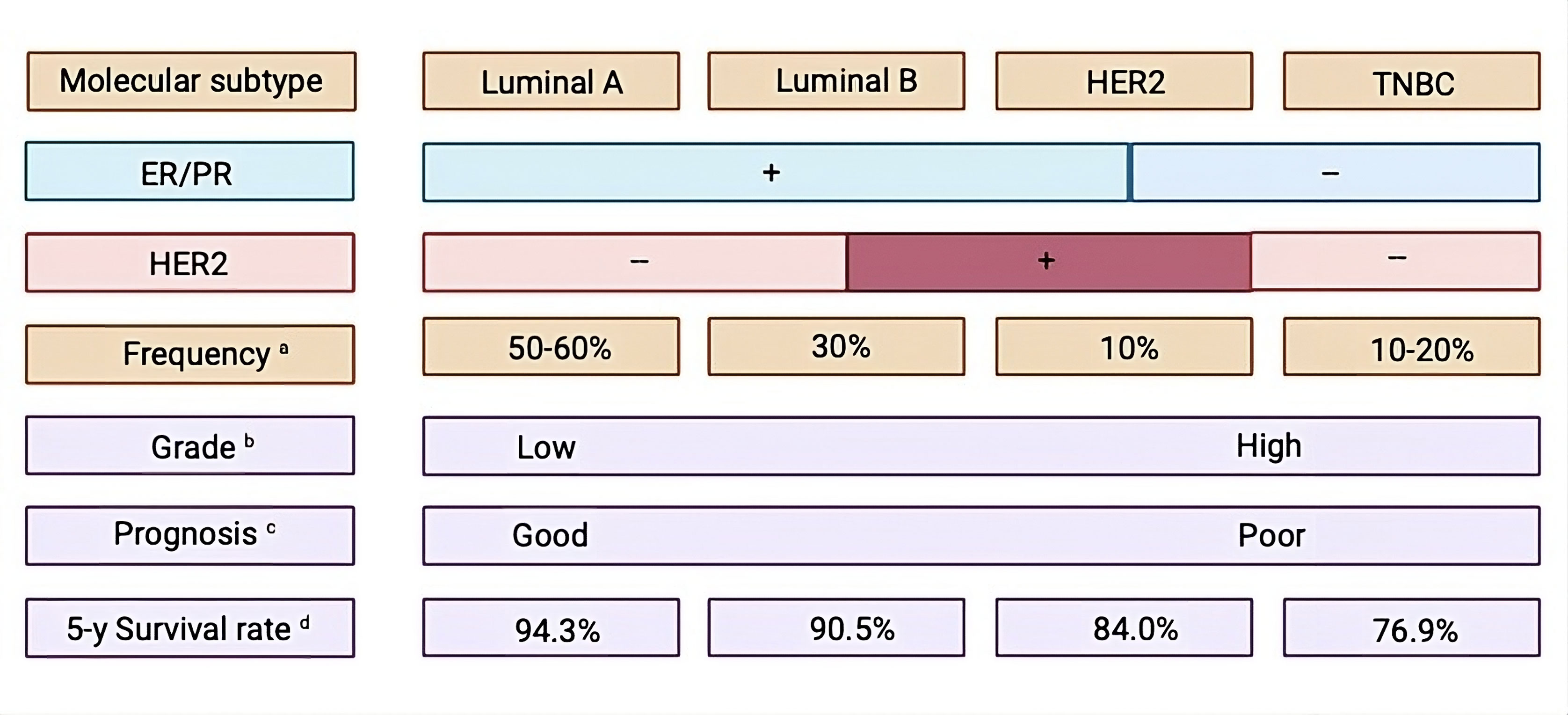
The origin of BCSCs remains a topic of active debate (Figure 2). One widely discussed hypothesis indicates that BCSCs may arise from normal mammary stem cells whose regular self-renewal processes have become deregulated. Evidence shows that normal mammary stem cells and BCSCs share several key traits, including telomerase activity, self-renewal capacity, differentiation ability, resistance to apoptosis, and the ability to migrate to specific locations [19]. Given that stem cells possess intrinsic mechanisms for self-renewal, they may need fewer mutations to sustain this ability than differentiated cells, which would require additional changes to activate self-renewal inappropriately [20]. Furthermore, the longer lifespan of mammary stem cells increases the likelihood of mutation accumulation, potentially leading these cells to moderately evolve into BCSCs. Another speculation proposes that BCSCs could originate from differentiated cells, though the underlying mechanism remains uncertain [21]. This was corroborated by Guo et al. (2012), who showed that the simultaneous transient expression of exogenous Slug and Sox9 was sufficient to transform differentiated luminal cells into mammary stem cells with the capacity to continuously replenish the mammary glands [22]. This notion was further reinforced by findings from Koren et al. (2015), which showed that when the mutation PIK3CAH1047R was expressed, lineage-committed basal Lgr5-positive and luminal keratin-8-positive cells in the adult mouse mammary gland dedifferentiated into a multipotent stem-like state [23].
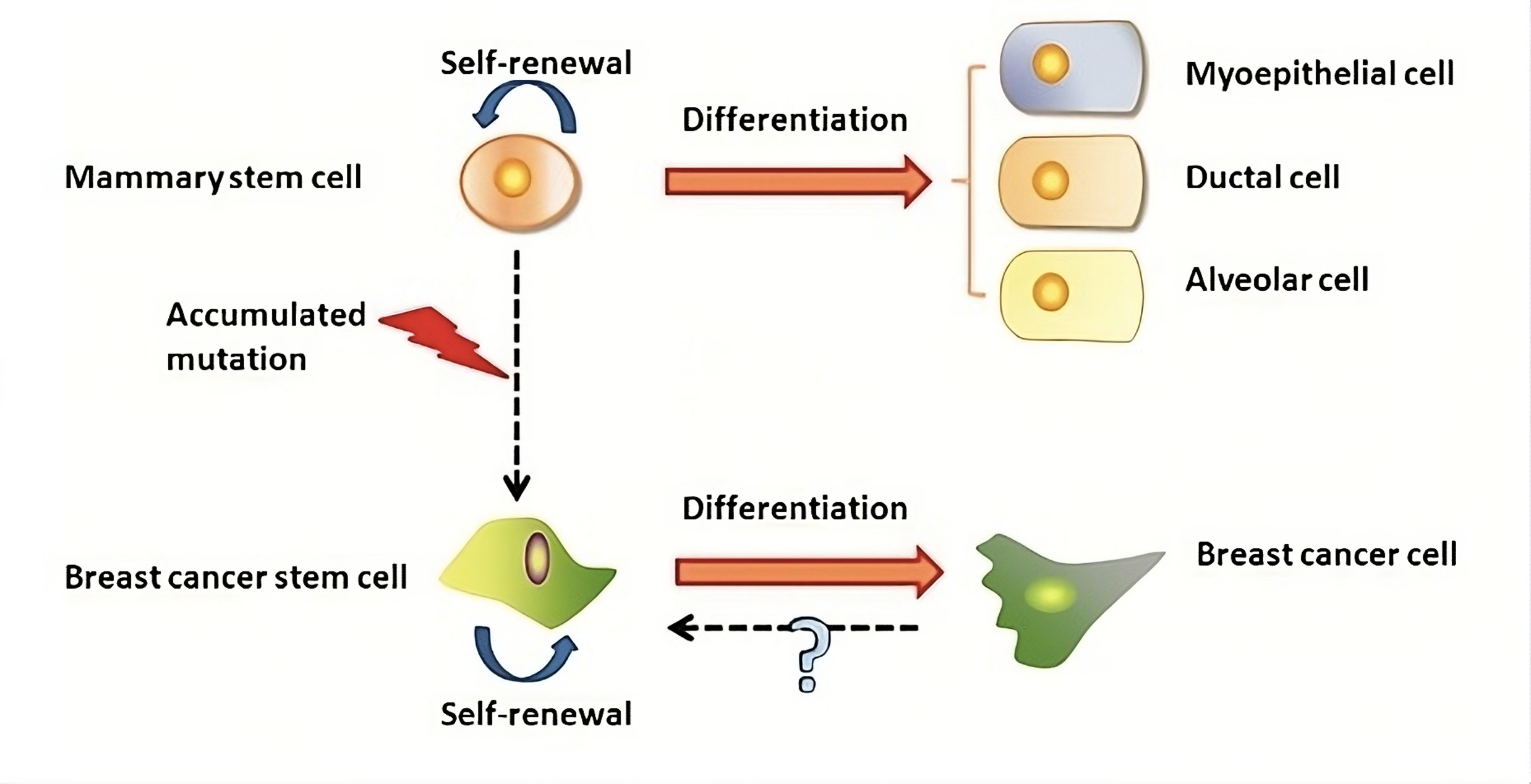
Maintaining the BCSC population requires CD44, a transmembrane glycoprotein that acts as a receptor for several growth factors and ligands in the extracellular matrix, to activate the Ras-MAPK and PI3K/AKT signaling pathways. Furthermore, CD44 triggers the p62-associated nuclear factor erythroid 2-related factor 2 (Nrf2) pathway, which shows the treatment resistance [33-35]. Increased radioresistance and worse prognoses have been associated with NF-κB activation and CD44 expression. The CD24-/Low phenotype further promotes resistance to therapy, even though the ALDH enzyme aids in the conversion of retinol to retinoic acid, a process required for the differentiation capacities of both normal and malignant stem cells [36].
BCSCs with the CD44+/CD24-/Low surface biomarker expression exhibit a more invasive, quiescent, and basal-like phenotype, whereas BCSCs with the ALDH1+ epithelial-like phenotype exhibit a more proliferative and localized luminal characteristic [37]. BCSCs expressing both CD44+/CD24-/Low and ALDH1+ markers are associated with enhanced tumorigenic and metastatic potential [38]. Additionally, BCSCs show increased expression of the CD44 variant isoform, CD44v6, which binds to key cytokines in the TME, promoting cancer progression, cell migration, and invasion [39]. CD133, CD49f, CD61, and CD29 are additional markers for BCSCs and are also present on normal mammary stem cells (MaSCs) (Figure 3). Combining these markers with CD44+/CD24-/Low/ALDH1+ expression provides a valuable approach for isolating BCSCs and may serve as useful prognostic indicators [40].
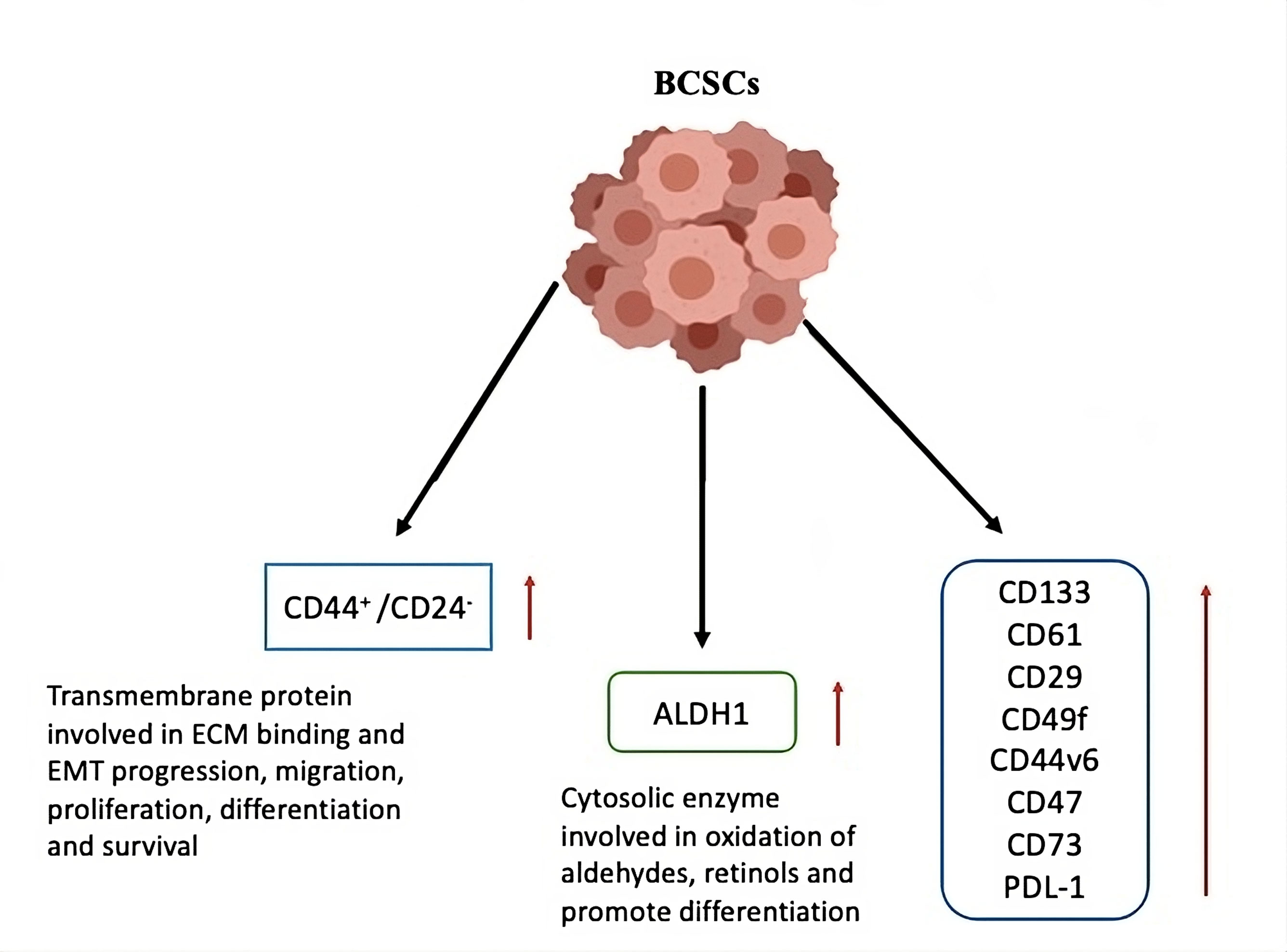
Cancer patients' risk assessment and treatment choices can be greatly improved by the creation of BCSC-based tools for prognosis prediction and practical application. Alternative biomarker-based techniques are to be investigated in light of the difficulties in acquiring serial samples from metastatic locations. For instance, some studies have shown that finding BCSC indicators in circulating tumor cells may serve as a stand-in for anti-CSC action [51]. In summary, designing new therapeutic strategies that specifically target CSCs could lead to more effective cancer treatments and help overcome therapy resistance.
Importantly, therapeutic targeting of BCSCs can be approached through various strategies, including targeting cell surface, cytoplasmic, and nuclear BCSC markers. Differentiation therapies and immunotherapy may also be effective ways to combat BCSCs' resistance to traditional medicines [52]. According to reviews by Saygin et al. [41] and Yang et al. [53], a number of BCSC-targeting treatments are presently undergoing clinical trials for different forms of cancer.
One ligand that has been found to specifically bind to CD44 is hyaluronic acid (HA) [56]. In contrast to normal tissues, BC cells have a noticeably increased absorption of HA, which is necessary to sustain high levels of P-glycoprotein (P-gp) production, a major cause of multidrug resistance [57]. Because of this property, HA is a desirable targeting moiety for anti-BCSC nanomedicines. Choleryl-hyaluronic acid (CHA) nanogel-drug conjugates were developed by Wei et al. (2013) with the express purpose of effectively treating cancer cells [58]. Through endocytosis mediated by the CD44 receptor, these nanogels were effectively ingested while concurrently engaging with the membrane of the cancer cell. Additionally, the cellular absorption of salinomycin (SLM) nanoparticles was increased by 1.5 times upon functionalization with HA coating [59].
Wang et al. (2013) developed a HA-mediated BCSC-targeting delivery system consisting of lipid bilayer-supported HA-MSS (Hyaluronan-modified mesoporous silicon nanoparticles) loaded with 8-hydroxyquinoline [60]. Their findings indicated that HA stimulated the adoption of HA-MSS in BCSCs. Furthermore, Ganesh et al. (2013) developed a number of HA-based self-assembling nanosystems that target CD44 for the delivery of siRNA. They identified increased gene silencing activity and siRNA delivery efficiency in drug-resistant tumor models with overexpressed CD44 receptors [61]. They discovered that siRNAs may be transfected into cancer cells with high CD44 expression using a variety of HA derivatives. It's interesting to note that high level of soluble HA may block CD44 receptors, which would limit cellular uptake by over 90% [61]. With the intention of targeting BCSCs, Chen et al. (2015) developed new multifunctionalized hyaluronan oligosaccharides-histidine-menthone 1,2-glycerol ketal (oHM) conjugates. Hyaluronan oligosaccharides are used by these oHM conjugates, which take the form of micelles, to bind selectively to the CD44 receptor found on BCSCs [62].
Chitosan’s chemical structure partially resembles that of hyaluronic acid (HA), but unlike HA, chitosan carries a positive charge, which may enhance the binding efficiency of chitosan-modified nanoparticles to mammalian cells. In order to specifically target CD44 receptors on BCSCs, Rao et al. (2015) developed doxorubicin-loaded polymeric nanoparticles coated with chitosan. When compared to the free drug, these tailored nanoparticles enhanced doxorubicin's ability to eradicate BCSCs by a factor of six [63]. With a focus on CD133 as a target receptor, Swaminathan et al. (2013) created polymeric nanoparticles modified with paclitaxel loaded anti-CD133 antibodies. Their study found that these nanoparticles effectively eliminated BCSCs in vitro and significantly decreased tumor formation in vivo, indicating that CD133 may be a promising target for anti-BCSC therapies [64].
For the active targeting of BCSCs, a number of other targets can be chosen in addition to the CD44 and CD133 receptors. BCSC population exhibits much higher expression of vasoactive intestinal peptide (VIP) receptors, which makes VIP a promising active targeting moiety for improving anti-BCSC efficacy [65]. It has been demonstrated that a new delivery system incorporating sterically stabilized curcumin phospholipid nanomicelles (C-SSM) conjugated with VIP efficiently suppresses the proliferation of BC cells that contain BCSCs [65].
Aydın (2013) used Herceptin (HER) as the targeted ligand for PLGA nanoparticles loaded with salinomycin (SAL), which enhanced the binding and accumulation of SAL in BC cells. Human epidermal growth factor receptor type 2 (HER2)-positive metastatic breast tumors, which overexpress HER2 in 25–30% of invasive breast cancers, can be treated with the authorized antibody HER. Targeting SAL to the bulk of BC may lead to a notable decrease in BCSCs because SAL suppresses the growth of BC and selectively removes human BCSCs from tumorspheres [66].
DNA repair genes are upregulated in BCSCs, along with improved reactive oxygen species (ROS) scavenging systems. The transcriptional profile of BCSCs extracted from the mammary glands of p53-null mice brought this to light [78]. These BCSC populations are crucial for the formation of chemoresistance, clonal evolution, and tumor progression after chemotherapy, and they have also been linked to resistance to platinum-based chemotherapy in a mouse mammary tumor model with a BRCA1/p53 mutation [79]. Autophagy has become a prominent response to chemotherapeutic drugs in CSCs throughout the last ten years. In order to maintain energy balance during nutritional shortage, stress, pathogen exposure, or hypoxia, autophagy was first discovered to be a catabolic process that controls cell survival and dormancy [80]. Later studies have linked autophagy to resistance to treatment in CSCs and tumor cells [81]. However, autophagy can have dual roles; it may suppress tumorigenesis in certain contexts while promoting it in others [82]. Autophagy has been shown to be vital for the tumorigenic capabilities of BCSCs [83] and is considered a key element of the pro-survival approaches employed by these cells, particularly in challenging tissue microenvironments associated with nutrient scarcity, cytotoxic treatments, and metastatic spread [82, 84]. The significance of autophagy in the survival strategies of BCSCs is particularly notable in the premetastatic phase, where disseminated BC cells can remain dormant for years or even decades before re-emerging as highly aggressive secondary tumors. Recent research has revealed that BCSCs are disseminated tumor cells (DTCs) with the capability to form recurrent metastatic lesions and survive metastatic dormancy [85]. Through a number of processes, including stimulation of autophagic pathways, BCSCs exhibit dormant phenotypes during metastatic latency, which is essential for their survival and capacity for metastasis [86]. Autophagy facilitates BCSC survival at metastatic sites by enabling these cells to evade apoptotic signals and withstand chemotherapeutic treatments. For instance, it increases p53 activity and DNA repair via autophagy-related 7 (ATG7) [88] and promotes SRC-mediated TRAIL resistance in bone metastases [87]. Additionally, new research has shown that the autophagy machinery keeps 6-phosphofructo-2-kinase/fructose-2,6-biphosphatase 3 (PFKFB3) expression low, which helps keep metastatic BC cells dormant. The aberrant expression of PFKFB3 in dormant BCSCs is restored by targeted reduction of autophagic factors that physically interact with PFKFB3, such as ATG3, ATG7, or p62/sequestosome-1. Proliferative pathways are reactivated as a result of this repair, and metastatic expansion follows [89]. Studies demonstrating the function of Spleen Tyrosine Kinase (SYK) in the epithelial-to-mesenchymal transition (EMT) required for BC metastasis have provided more proof of connection between autophagy and metastatic dormancy. It was discovered that SYK which is located within cytoplasmic RNA processing structures called P-bodies, is significantly expressed in cells going through EMT. During the mesenchymal-to-epithelial transition (MET), autophagy-mediated P-body clearance depends on SYK activity. By preventing the clearance of P-bodies and MET, either pharmacologically blocking SYK with fostamatinib or genetically knocking out ATG7 inhibited the growth of metastatic tumors [90].
Autophagy affects tumor immunosurveillance in a number of ways as well as controlling the plasticity and dormancy of BCSCs. On the one hand, autophagy can reduce the anti-tumor immune responses that are mediated by CTLs and NK cells [91]. On the other hand, it may be involved in the development of certain immune cells and is essential for antigen presentation to T cells [91]. Together with the limited potency and effectiveness of many investigated anti-autophagic drugs, this dual role of autophagy in tumor immune modulation may help to explain the difficulties encountered in the clinical usage of autophagy inhibitors [92]. Finally, hypoxia signaling and the hypoxia-induced dormancy pathway in BC are intimately linked to autophagy. Chemotherapy can also cause hypoxic reactions, which set off a chain of events that increases BCSCs by releasing calcium from the endoplasmic reticulum and upregulating pluripotency genes [93].
None.
Availability of data and materials
Data and materials are available on request from the authors.
Ethical policy
Not applicable.
Author contributions
BC contributed to draft, critical revision of the article and approved the final submission; MWA contributed to the revision and figure production.
I declare that there is no conflict of interest regarding the publication of this document.
Funding
None.
- Siegel RL, Miller KD, Jemal A: Cancer statistics, 2018. CA Cancer J Clin 2018, 68(1): 7-30.
- Sin WC, Lim CL: Breast cancer stem cells-from origins to targeted therapy. Stem Cell Investig 2017, 4: 96.
- Toss A, Cristofanilli M: Molecular characterization and targeted therapeutic approaches in breast cancer. Breast Cancer Res 2015, 17(1): 60.
- Nielsen TO, Hsu FD, Jensen K, Cheang M, Karaca G, Hu Z, Hernandez-Boussard T, Livasy C, Cowan D, Dressler L et al: Immunohistochemical and clinical characterization of the basal-like subtype of invasive breast carcinoma. Clin Cancer Res 2004, 10(16): 5367-5374.
- Perou CM, Sørlie T, Eisen MB, Van De Rijn M, Jeffrey SS, Rees CA, Pollack JR, Ross DT, Johnsen H, Akslen LA et al: Molecular portraits of human breast tumours. Nature 2000, 406(6797): 747-752.
- Dawson SJ, Provenzano E, Caldas C: Triple negative breast cancers: clinical and prognostic implications. Eur J Cancer 2009, 45(1): 27-40.
- Cancer Genome Atlas Network: Comprehensive molecular portraits of human breast tumours. Nature 2012, 490(7418): 61-70.
- Bertucci F, Ng CK, Patsouris A, Droin N, Piscuoglio S, Carbuccia N, Soria JC, Dien AT, Adnani Y, Kamal M et al: Genomic characterization of metastatic breast cancers. Nature 2019, 569(7757): 560-564.
- Polyak K: Heterogeneity in breast cancer. J Clin Invest 2011, 121(10): 3786-3788.
- Kim C, Gao R, Sei E, Brandt R, Hartman J, Hatschek T, Crosetto N, Foukakis T, Navin NE: Chemoresistance evolution in triple-negative breast cancer delineated by single-cell sequencing. Cell 2018, 173(4): 879-893.
- De Angelis ML, Francescangeli F, La Torre F, Zeuner A: Stem cell plasticity and dormancy in the development of cancer therapy resistance. Front Oncol 2019, 9: 626.
- Tang DG: Understanding cancer stem cell heterogeneity and plasticity. Cell Res 2012, 22(3): 457-472.
- Prager BC, Xie Q, Bao S, Rich JN: Cancer stem cells: the architects of the tumor ecosystem. Cell Stem Cell 2019, 24(1): 41-53.
- De Angelis ML, Francescangeli F, Zeuner A: Breast cancer stem cells as drivers of tumor chemoresistance, dormancy and relapse: new challenges and therapeutic opportunities. Cancers 2019, 11(10): 1569.
- Polyak K: Breast cancer: origins and evolution. J Clin Invest 2007, 117(11): 3155-3163.
- Williams C, Helguero L, Edvardsson K, Haldosén LA, Gustafsson JÅ: Gene expression in murine mammary epithelial stem cell-like cells shows similarities to human breast cancer gene expression. Breast Cancer Res 2009, 11(3): R26.
- Liu S, Dontu G, Wicha MS: Mammary stem cells, self-renewal pathways, and carcinogenesis. Breast Cancer Res 2005, 7(3): 86-95.
- Wicha MS, Dontu G, Al-Hajj M, Clarke MF: Stem cells in normal breast development and breast cancer. Breast Cancer Res 2003, 5(Suppl 1): 59-72.
- Dontu G, El-Ashry D, Wicha MS: Breast cancer, stem/progenitor cells and the estrogen receptor. Trends Endocrinol Metab 2004, 15(5): 193-197.
- Passegué E, Jamieson CH, Ailles LE, Weissman IL: Colloquium Paper: Regenerative Medicine: Normal and leukemic hematopoiesis: Are leukemias a stem cell disorder or a reacquisition of stem cell characteristics? Proc Natl Acad Sci USA 2003, 100(Suppl 1): 11842-11849.
- Lu B, Huang X, Mo J, Zhao W: Drug delivery using nanoparticles for cancer stem-like cell targeting. Front Pharmacol 2016, 7: 84.
- Guo W, Keckesova Z, Donaher JL, Shibue T, Tischler V, Reinhardt F, Itzkovitz S, Noske A, Zürrer-Härdi U, Bell G et al: Slug and Sox9 cooperatively determine the mammary stem cell state. Cell 2012, 148(5): 1015-1028.
- Koren S, Reavie L, Couto JP, De Silva D, Stadler MB, Roloff T, Britschgi A, Eichlisberger T, Kohler H, Aina O et al: PIK3CA H1047R induces multipotency and multi-lineage mammary tumours. Nature 2015, 525(7567): 114-118.
- Bozorgi A, Khazaei M, Khazaei MR: New findings on breast cancer stem cells: a review. J Breast Cancer 2015, 18(4): 303-312.
- Korkaya H, Liu S, Wicha MS: Regulation of cancer stem cells by cytokine networks: attacking cancer's inflammatory roots. Clin Cancer Res 2011, 17(19): 6125-9.
- Guagnano V, Kauffmann A, Wöhrle S, Stamm C, Ito M, Barys L, Pornon A, Yao Y, Li F, Zhang Y et al: FGFR genetic alterations predict for sensitivity to NVP-BGJ398, a selective pan-FGFR inhibitor. Cancer Discov 2012, 2(12): 1118-33.
- Ginestier C, Liu S, Diebel ME, Korkaya H, Luo M, Brown M, Wicinski J, Cabaud O, Charafe-Jauffret E, Birnbaum D et al: CXCR1 blockade selectively targets human breast cancer stem cells in vitro and in xenografts. J Clin Invest 2010, 120(2): 485-497.
- Chapellier M, Maguer-Satta V: BMP2, a key to uncover luminal breast cancer origin linked to pollutant effects on epithelial stem cells niche. Mol Cell Oncol 2016, 3(3): e1026527.
- LaBarge MA: The difficulty of targeting cancer stem cell niches. Clin Cancer Res 2010, 16(12): 3121-3129.
- Al-Hajj M, Wicha MS, Benito-Hernandez A, Morrison SJ, Clarke MF: Prospective identification of tumorigenic breast cancer cells. Proc Natl Acad Sci 2003, 100(7): 3983-3988.
- Kai K, Arima Y, Kamiya T, Saya H: Breast cancer stem cells. Breast Cancer 2010, 17(2): 80-85.
- Liu R, Wang X, Chen GY, Dalerba P, Gurney A, Hoey T, Sherlock G, Lewicki J, Shedden K, Clarke MF et al: The prognostic role of a gene signature from tumorigenic breast-cancer cells. New Engl J Med 2007, 356(3): 217-226.
- Al-Othman N, Ahram M, Alqaraleh M: Role of androgen and microRNA in triple-negative breast cancer. Breast Dis 2020, 39(1): 1-13.
- Santamaria‐Martínez A, Huelsken J: The niche under siege: novel targets for metastasis therapy. J Intern Med 2013, 274(2): 127-136.
- Dufraine J, Funahashi Y, Kitajewski J: Notch signaling regulates tumor angiogenesis by diverse mechanisms. Oncogene 2008, 27(38): 5132-5137.
- Chute JP, Muramoto GG, Whitesides J, Colvin M, Safi R, Chao NJ, McDonnell DP: Inhibition of aldehyde dehydrogenase and retinoid signaling induces the expansion of human hematopoietic stem cells. Proc Natl Acad Sci 2006, 103(31): 11707-11712.
- LeBedis C, Chen K, Fallavollita L, Boutros T, Brodt P: Peripheral lymph node stromal cells can promote growth and tumorigenicity of breast carcinoma cells through the release of IGF‐I and EGF. Int J Cancer 2002, 100(1): 2-8.
- Croker AK, Goodale D, Chu J, Postenka C, Hedley BD, Hess DA, Allan AL: High aldehyde dehydrogenase and expression of cancer stem cell markers selects for breast cancer cells with enhanced malignant and metastatic ability. J Cell Mol Med 2009, 13(8b): 2236-2252.
- Snyder EL, Bailey D, Shipitsin M, Polyak K, Loda M: Identification of CD44v6+/CD24− breast carcinoma cells in primary human tumors by quantum dot-conjugated antibodies. Lab Invest 2009, 89(8): 857-866.
- Taurin S, Alkhalifa H: Breast cancers, mammary stem cells, and cancer stem cells, characteristics, and hypotheses. Neoplasia 2020, 22(12): 663-678.
- Saygin C, Matei D, Majeti R, Reizes O, Lathia JD: Targeting cancer stemness in the clinic: from hype to hope. Cell Stem Cell 2019, 24(1): 25-40.
- Shiozawa Y, Nie B, Pienta KJ, Morgan TM, Taichman RS: Cancer stem cells and their role in metastasis. Pharmacol Ther 2013, 138(2): 285-293.
- Lee HE, Kim JH, Kim YJ, Choi SY, Kim SW, Kang E, Chung IY, Kim IA, Kim EJ, Choi Y et al: An increase in cancer stem cell population after primary systemic therapy is a poor prognostic factor in breast cancer. Br J Cancer 2011, 104(11): 1730-1738.
- Creighton CJ, Li X, Landis M, Dixon JM, Neumeister VM, Sjolund A, Rimm DL, Wong H, Rodriguez A, Herschkowitz JI et al: Residual breast cancers after conventional therapy display mesenchymal as well as tumor-initiating features. Proc Natl Acad Sci 2009, 106(33): 13820-13825.
- Abraham BK, Fritz P, McClellan M, Hauptvogel P, Athelogou M, Brauch H: Prevalence of CD44+/CD24−/low cells in breast cancer may not be associated with clinical outcome but may favor distant metastasis. Clinical Cancer Res 2005, 11(3): 1154-1159.
- Yu F, Yao H, Zhu P, Zhang X, Pan Q, Gong C, Huang Y, Hu X, Su F, Lieberman J et al: let-7 regulates self renewal and tumorigenicity of breast cancer cells. Cell 2007, 131(6): 1109-1123.
- Marcato P, Dean CA, Pan D, Araslanova R, Gillis M, Joshi M, Helyer L, Pan L, Leidal A, Gujar S et al: Aldehyde dehydrogenase activity of breast cancer stem cells is primarily due to isoform ALDH1A3 and its expression is predictive of metastasis. Stem Cells 2011, 29(1): 32-45.
- Charafe-Jauffret E, Ginestier C, Iovino F, Tarpin C, Diebel M, Esterni B, Houvenaeghel G, Extra JM, Bertucci F, Jacquemier J et al: Aldehyde dehydrogenase 1–Positive cancer stem cells mediate metastasis and poor clinical outcome in inflammatory breast cancer. Clin Cancer Res 2010, 16(1): 45-55.
- Dionísio MR, Vieira AF, Carvalho R, Conde I, Oliveira M, Gomes M, Pinto MT, Pereira P, Pimentel J, Souza C et al: BR-BCSC signature: the cancer stem cell profile enriched in brain metastases that predicts a worse prognosis in lymph node-positive breast cancer. Cells 2020, 9(11): 2442.
- Liu R, Wang X, Chen GY, Dalerba P, Gurney A, Hoey T, Sherlock G, Lewicki J, Shedden K, Clarke MF et al: The prognostic role of a gene signature from tumorigenic breast-cancer cells. N Engl J Med 2007, 356(3): 217-226.
- Zeng X, Liu C, Yao J, Wan H, Wan G, Li Y, Chen N: Breast cancer stem cells, heterogeneity, targeting therapies and therapeutic implications. Pharmacol Res 2021, 163: 105320.
- Zeng X, Liu C, Yao J, Wan H, Wan G, Li Y, Chen N: Breast cancer stem cells, heterogeneity, targeting therapies and therapeutic implications. Pharmacol Res 2021, 163: 105320.
- Yang L, Shi P, Zhao G, Xu J, Peng W, Zhang J, Zhang G, Wang X, Dong Z, Chen F et al: Targeting cancer stem cell pathways for cancer therapy. Signal Transduct Target Ther 2020, 5(1): 8.
- Aires A, Ocampo SM, Simões BM, Rodríguez MJ, Cadenas JF, Couleaud P, Spence K, Latorre A, Miranda R, Somoza Á et al: Multifunctionalized iron oxide nanoparticles for selective drug delivery to CD44-positive cancer cells. Nanotechnology 2016, 27(6): 065103.
- Gener P, Gouveia LP, Sabat GR, de Sousa Rafael DF, Fort NB, Arranja A, Fernández Y, Prieto RM, Ortega JS, Arango D et al: Fluorescent CSC models evidence that targeted nanomedicines improve treatment sensitivity of breast and colon cancer stem cells. Nanomedicine 2015, 11(8): 1883-1892.
- Shen S, Xia JX, Wang J: Nanomedicine-mediated cancer stem cell therapy. Biomaterials 2016, 74: 1-18.
- Gotte M, Yip GW: Heparanase, hyaluronan, and CD44 in cancers: a breast carcinoma perspective. Cancer Res 2006, 66(21): 10233-10237.
- Wei X, Senanayake TH, Warren G, Vinogradov SV: Hyaluronic acid-based nanogel–drug conjugates with enhanced anticancer activity designed for the targeting of CD44-positive and drug-resistant tumors. Bioconjug Chem 2013, 24(4): 658-668.
- Muntimadugu E, Kumar R, Saladi S, Rafeeqi TA, Khan W: CD44 targeted chemotherapy for co-eradication of breast cancer stem cells and cancer cells using polymeric nanoparticles of salinomycin and paclitaxel. Colloids Surf B Biointerfaces 2016, 143: 532-546.
- Wang D, Huang J, Wang X, Yu Y, Zhang H, Chen Y, Liu J, Sun Z, Zou H, Sun D et al: The eradication of breast cancer cells and stem cells by 8-hydroxyquinoline-loaded hyaluronan modified mesoporous silica nanoparticle-supported lipid bilayers containing docetaxel. Biomaterials 2013, 34(31): 7662-7673.
- Ganesh S, Iyer AK, Morrissey DV, Amiji MM: Hyaluronic acid based self-assembling nanosystems for CD44 target mediated siRNA delivery to solid tumors. Biomaterials 2013, 34(13): 3489-3502.
- Chen D, Wang G, Song W, Zhang Q: Novel CD44 receptor targeting multifunctional “nano-eggs” based on double pH-sensitive nanoparticles for co-delivery of curcumin and paclitaxel to cancer cells and cancer stem cells. J Nanopart Res 2015, 17: 1-10.
- Rao W, Wang H, Han J, Zhao S, Dumbleton J, Agarwal P, Zhang W, Zhao G, Yu J, Zynger DL et al: Chitosan-decorated doxorubicin-encapsulated nanoparticle targets and eliminates tumor reinitiating cancer stem-like cells. ACS Nano 2015, 9(6): 5725-5740.
- Swaminathan SK, Roger E, Toti U, Niu L, Ohlfest JR, Panyam J: CD133-targeted paclitaxel delivery inhibits local tumor recurrence in a mouse model of breast cancer. J Control Release 2013, 171(3): 280-287.
- Gülçür E, Thaqi M, Khaja F, Kuzmis A, Önyüksel H: Curcumin in VIP-targeted sterically stabilized phospholipid nanomicelles: a novel therapeutic approach for breast cancer and breast cancer stem cells. Drug Deliv Transl Res 2013, 3(6): 562-574.
- Aydın RS: Herceptin‐decorated salinomycin‐loaded nanoparticles for breast tumor targeting. J Biomed Res A 2013, 101(5): 1405-1415.
- Nobili S, Lapucci A, Landini I, Coronnello M, Roviello G, Mini E: Role of ATP-binding cassette transporters in cancer initiation and progression. Semin Cancer Biol 2020, 60: 72-95.
- Begicevic RR, Falasca M: ABC transporters in cancer stem cells: beyond chemoresistance. Int J Mol Sci 2017, 18(11): 2362.
- Britton K, Eyre R, Harvey IJ, Stemke-Hale K, Browell D, Lennard TW, Meeson A: Breast cancer, side population cells and ABCG2 expression. Cancer Lett 2012, 323(1): 97-105.
- Mukherjee P, Gupta A, Chattopadhyay D, Chatterji U: Modulation of SOX2 expression delineates an end-point for paclitaxel-effectiveness in breast cancer stem cells. Sci Rep 2017, 7(1): 9170.
- Ginestier C, Hur MH, Charafe-Jauffret E, Monville F, Dutcher J, Brown M, Jacquemier J, Viens P, Kleer CG, Liu S et al: ALDH1 is a marker of normal and malignant human mammary stem cells and a predictor of poor clinical outcome. Cell Stem cell 2007, 1(5): 555-567.
- Croker AK, Goodale D, Chu J, Postenka C, Hedley BD, Hess DA, Allan AL: High aldehyde dehydrogenase and expression of cancer stem cell markers selects for breast cancer cells with enhanced malignant and metastatic ability. J Cell Mol Med 2009, 13(8b): 2236-2252.
- Croker AK, Allan AL: Inhibition of aldehyde dehydrogenase (ALDH) activity reduces chemotherapy and radiation resistance of stem-like ALDH hi CD44+ human breast cancer cells. Breast Cancer Res Treat 2012, 133(1): 75-87.
- Kida K, Ishikawa T, Yamada A, Shimada K, Narui K, Sugae S, Shimizu D, Tanabe M, Sasaki T, Ichikawa Y et al: Effect of ALDH1 on prognosis and chemoresistance by breast cancer subtype. Breast Cancer Res Treat 2016, 156(2): 261-269.
- Phillips TM, McBride WH, Pajonk F: The response of CD24−/low/CD44+ breast cancer–initiating cells to radiation. J Natl Cancer Inst 2006, 98(24): 1777-1785.
- Diehn M, Cho RW, Lobo NA, Kalisky T, Dorie MJ, Kulp AN, Qian D, Lam JS, Ailles LE, Wong M et al: Association of reactive oxygen species levels and radioresistance in cancer stem cells. Nature 2009, 458(7239): 780-783.
- Del Vecchio CA, Feng Y, Sokol ES, Tillman EJ, Sanduja S, Reinhardt F, Gupta PB: De-differentiation confers multidrug resistance via noncanonical PERK-Nrf2 signaling. PLoS Biol 2014, 12(9): e1001945.
- Zhang M, Behbod F, Atkinson RL, Landis MD, Kittrell F, Edwards D, Medina D, Tsimelzon A, Hilsenbeck S, Green JE et al: Identification of tumor-initiating cells in a p53-null mouse model of breast cancer. Cancer Res 2008, 68(12): 4674-4682.
- Shafee N, Smith CR, Wei S, Kim Y, Mills GB, Hortobagyi GN, Stanbridge EJ, Lee EY: Cancer stem cells contribute to cisplatin resistance in Brca1/p53–mediated mouse mammary tumors. Cancer Res 2008, 68(9): 3243-3250.
- Dikic I, Elazar Z: Mechanism and medical implications of mammalian autophagy. Nat Rev Mol Cell Biol 2018, 19(6): 349-364.
- Smith AG, Macleod KF: Autophagy, cancer stem cells and drug resistance. J Pathol 2019, 247(5): 708-718.
- Flynn AL, Schiemann WP: Autophagy in breast cancer metastatic dormancy: Tumor suppressing or tumor promoting functions? J Cancer Metast Treat 2019, 5: 43.
- Gong C, Bauvy C, Tonelli G, Yue W, Delomenie C, Nicolas V, Zhu Y, Domergue V, Marin-Esteban V, Tharinger H et al: Beclin 1 and autophagy are required for the tumorigenicity of breast cancer stem-like/progenitor cells. Oncogene 2013, 32(18): 2261-2272.
- Yeo SK, Wen J, Chen S, Guan JL: Autophagy differentially regulates distinct breast cancer stem-like cells in murine models via EGFR/Stat3 and Tgfβ/Smad signaling. Cancer Res 2016, 76(11): 3397-3410.
- Carcereri de Prati A, Butturini E, Rigo A, Oppici E, Rossin M, Boriero D, Mariotto S: Metastatic breast cancer cells enter into dormant state and express cancer stem cells phenotype under chronic hypoxia. J Cell Biochem 2017, 118(10): 3237-3248.
- Vera-Ramirez L, Vodnala SK, Nini R, Hunter KW, Green JE: Autophagy promotes the survival of dormant breast cancer cells and metastatic tumour recurrence. Nature commun 2018, 9(1): 1944.
- Zhang XH, Wang Q, Gerald W, Hudis CA, Norton L, Smid M, Foekens JA, Massagué J: Latent bone metastasis in breast cancer tied to Src-dependent survival signals. Cancer Cell 2009, 16(1): 67-78.
- Lee IH: Atg7 modulates p53 activity to regulate cell cycle and survival during metabolic stress. Science 2012, 336(6068): 225-228.
- La Belle Flynn A, Calhoun BC, Sharma A, Chang JC, Almasan A, Schiemann WP: Autophagy inhibition elicits emergence from metastatic dormancy by inducing and stabilizing Pfkfb3 expression. Nature Commun 2019, 10(1): 3668.
- Shinde A, Hardy SD, Kim D, Akhand SS, Jolly MK, Wang WH, Anderson JC, Khodadadi RB, Brown WS, George JT et al: Spleen tyrosine kinase-mediated autophagy is required for epithelial–mesenchymal plasticity and metastasis in breast cancer. Cancer Res 2019, 79(8): 1831-1843.
- Janji B, Viry E, Moussay E, Paggetti J, Arakelian T, Mgrditchian T, Messai Y, Noman MZ, Van Moer K, Hasmim M et al: The multifaceted role of autophagy in tumor evasion from immune surveillance. Oncotarget 2016, 7(14): 17591-17607.
- Guntuku L, Gangasani JK, Thummuri D, Borkar RM, Manavathi B, Ragampeta S, Vaidya JR, Sistla R, Vegi NG: IITZ-01, a novel potent lysosomotropic autophagy inhibitor, has single-agent antitumor efficacy in triple-negative breast cancer in vitro and in vivo. Oncogene 2019, 38(4): 581-595.
- Lu H, Chen I, Shimoda LA, Park Y, Zhang C, Tran L, Zhang H, Semenza GL: Chemotherapy-induced Ca2+ release stimulates breast cancer stem cell enrichment. Cell Rep 2017, 18(8): 1946-1957.
Asia-Pacific Journal of Oncology
print ISSN: 2708-7980, online ISSN: 2708-7999
Copyright © Asia Pac J Oncol. This work is licensed under a Creative Commons Attribution-NonCommercial-No Derivatives 4.0 International (CC BY-NC-ND 4.0) License.