Review Article | Open Access
A mini-review on metal-based breakthroughs in photodynamic therapy
S. Dhinesh Kumar1, Kaaviya. J1
1Department of Pharmaceutical Chemistry, KMCH College Of Pharmacy, Affiliated to the Tamil Nadu Dr. M.G.R Medical University, Coimbatore, Tamil Nadu, India.
Correspondence: Kaaviya. J (Department of Pharmaceutical Chemistry, KMCH College of Pharmacy, Kovai estate, Kalapatti road, Coimbatore 641048, Tamil Nadu, India; Email: dhineshsasi13@gmail.com).
Asia-Pacific Journal of Oncology 2023, 4: 17-21. https://doi.org/10.32948/ajo.2023.11.28
Received: 18 Nov 2023 | Accepted: 29 Nov 2023 | Published online: 03 Dec 2023
Key words photodynamic therapy, photosensitizers, metal-organic framework, reactive oxygen species
Although it has achieved noteworthy success, the currently employed clinical photosensitizers exhibit certain shortcomings. In response, a growing body of research has been dedicated to enhancing their performance through the introduction of metal ions. Addressing the imperative for improved PDT efficacy, considerable focus is being directed towards innovative designs of PS frameworks. This study aims to consolidate the understanding of PDT while outlining forthcoming progress aimed at augmenting therapeutic efficiency within clinical trials [4].
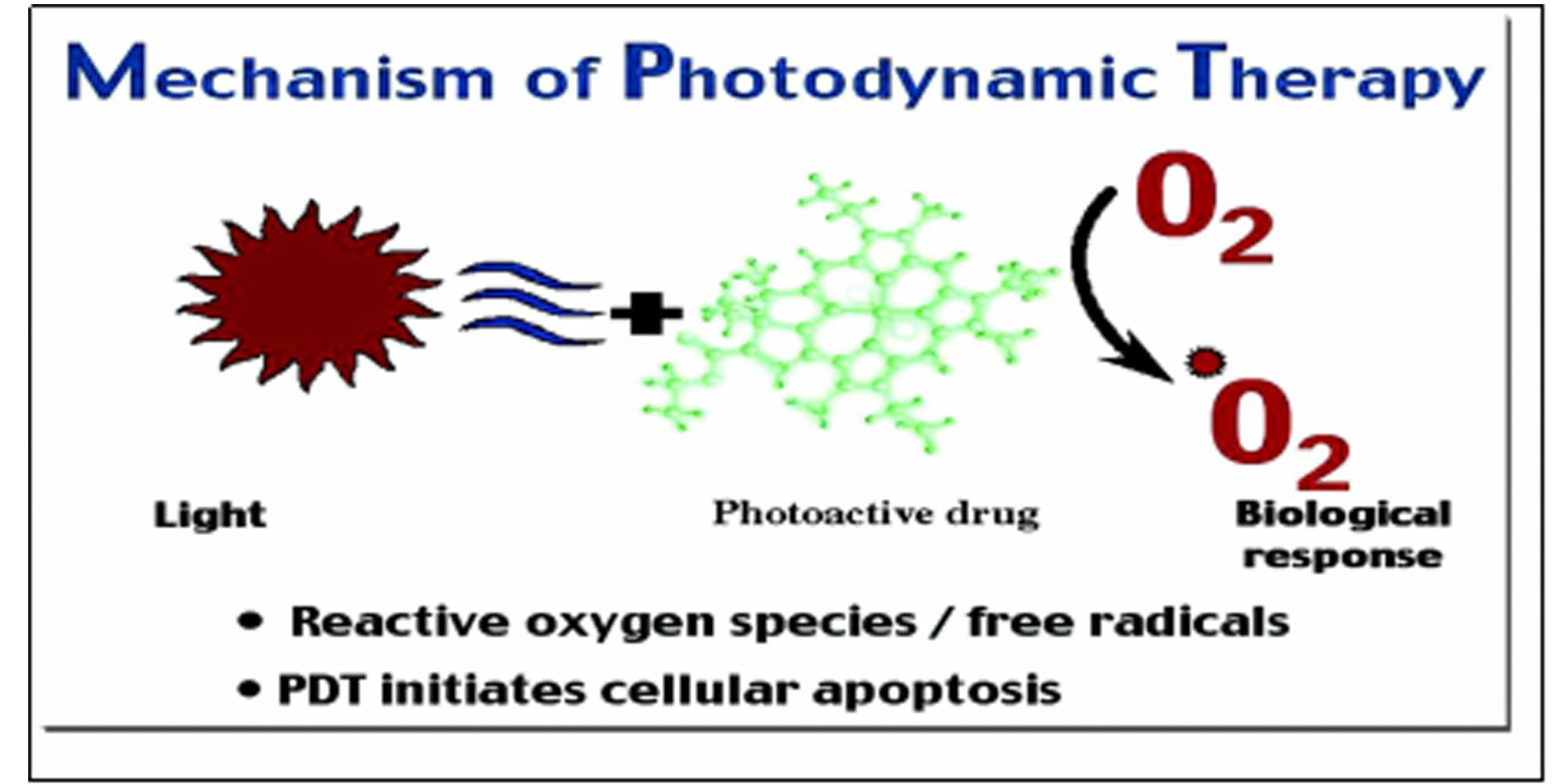
Cellular demise: Apoptosis and necrosis
As given in the following Figure 1, with the light irradiation of the Photosentizers, the generation of ROS is initiated. These oxygen-derived radicals infiltrate the body’s tumor cells, inducing their demise through a combination of apoptosis and necrosis pathways [7].
Impairing vascular dynamics
The oxygen radicals produced exert their impact on tumor vasculature, disrupting its integrity and leading to a deficiency in oxygen and nutrient supply to the affected cells. Consequently, a hypoxic environment ensues within the tumor, ultimately culminating in cellular death.
Provoking immunological response
Photodynamic therapy has the potential to elicit an inflammatory reaction that activates an immune response targeting the malignant cells. This triggered immune cascade serves as a potent mechanism for eliminating cancerous cells, further enhancing the therapeutic efficacy of the treatment.
Photosensitizers
Photosensitizers are safe molecules upon administration, accumulate within tumor cells, and upon exposure to specific wavelengths of light, trigger the generation of oxygen radicals. Phototfrin marked a historic milestone, becoming the first globally approved PS for esophageal and lung cancer treatment, and its application extended to diverse malignant and non-malignant conditions [9]. However, the treatment effectiveness faces challenges due to insufficient light penetration and skin photosensitivity caused by Photofrin. Sequentially, 5-aminolevulinic acid (Levulen), Temoporfin (Foscon), Verteporfin (Visudyne), Telaporfin (Foscon), and LUZ111 (Redaporfin) emerged as successive PSs for clinical PDT use [10]. Yet, these PSs share common limitations, including intricate synthesis/purification processes, limited water solubility, photostability concerns, inadequate cancer selectivity, and slow body clearance, leading to reduced ROS generation efficiency during PDT. This underscores a pressing need for enhancing existing compounds and designing novel Photosensitizers with more efficient architectural configurations [11].
Currently, Photodynamic therapy and photosensitizers exhibit diverse molecular structures, categorized into three generational tiers. The initial generation encompasses water-soluble PSs such as porfimer sodium and Heamatoporphyrin derivative (HpD) [12]. Evolving in the second generation, the PS derivatives derived from chlorins, bacteriochlorins, and phthalocyanines exhibit enhanced efficacy in cancerous regions due to their potent absorbance properties, enabling greater light penetration. The third generation includes PS molecules with heightened tumor selectivity, achieved by either amalgamation with targeting entities or encapsulation with carriers. Notably metal-complexes-based PSs, notably those containing metals like Sn, Lu, and Pd, represent a staple in the clinical development of PDT, showcasing improved efficacy and selectivity [13].
(A) Metal complexes in photosensitizers: Recently significant attention has been directed towards the potential utilization of transition metal coordination complexes and organic fluorophores as effective Photosensitizers in PDT. Transition metal complexes, exemplified by ruthenium (II) and iridium (III) complexes, alongside polymetallic counterparts, have emerged as notable contenders in the realms of therapeutics and bioimaging [14]. Renowned for their structural adaptability, exceptional photostability, sizeable Stokes shifts, prolonged emissions duration, and elevated singlet oxygen yields, these complexes also boast attributes such as extensive two-/multi- photon absorption, noteworthy cellular uptake, and precise organelle targeting capabilities. The convergence of these favorable properties has garnered considerable interest among researchers, signaling a promising avenue for exploration [15, 16]. The integration of transition metal complexes into Photosensitizers extends the duration of excited state interactions with molecular oxygen. This effect seems from the substantial atomic mass and larger atomic dimensions of these complexes. Notably, their straightforward synthesis results in Photosensitizers characterized by exceptional selectivity and specificity. Furthermore, the presence of metal ions contributes to improved PDT efficacy, as evidenced by heightened light absorption at relatively longer wavelengths and a substantial molar extinction coefficient [17].
(B) Nanomaterials in the photosensitizers: Introducing a new frontier in nanomaterials, Metal-Organic Frameworks (MOFs) have emerged, boasting an array of merits encompassing high porosity, expensive specific surface area, modifiable pore dimensions, and facile functionalization. These attributes render MOFs adept carriers for encapsulating Photosensitizer or enhancing Photosensitizer accumulation within targeted cells during PDT. In parallel, the ascent of organic fluorophore-based Photosensitizer brings forth attributes of low toxicity, commendable biocompatibility, and enduring triplet lifetimes. Significantly, the fluorescence emitted by these PSs during PDT enables real-time treatment monitoring. In recent times, propelled by nanotechnology’s progression, a tapestry of supramolecular nanoparticles, liposomes, metal-organic frameworks, and 2D nanosheets [18] has been woven, intricately enhancing the safety and efficacy of the Photosensitizer. This burgeoning array of nanomaterials revolutionizes aspects such as biocompatibility, precision targeting, controlled release, and pharmacokinetics. Yet, amidst this advancement, the biosafety evaluation of metal-complex-functionalized nanomaterials remains an essential endeavor [19]. A comprehensive scrutiny of the long-term consequences – ranging from biocompatibility and pharmacokinetic profiles to systemic toxicities, biological metabolic pathways, and immunogenicity – must be meticulously undertaken in animal models before embarking on the path of further clinical exploration and application. With a forward gaze, the strategic utilization of metal-complex-functionalized nanomaterials unfurls a vista of prospective applications, poised to redefine the diagnosis and treatment landscape across various diseases, notably within the realm of cancer therapy [20].
PDT functions through multifaceted mechanisms, principally utilizing photosensitizers that, upon exposure to tailored light wavelengths, instigate the production of Reactive Oxygen Species (ROS). These ROS infiltrate tumor cells, triggering cellular demise through a complex interplay of apoptosis and necrosis pathways. Beyond cellular destruction, PDT disrupts tumor vasculature, leading to oxygen and nutrient deprivation within the tumor microenvironment, ultimately causing cellular demise. Furthermore, PDT has the remarkable capability to incite an immune response, bolstering its therapeutic efficacy by marshaling the immune system against malignant cells.
Recent strides focus intensely on refining photosensitizer efficacy and specificity. Notably, metal-complex-based photosensitizers, typified by transition metal coordination complexes, demonstrate extraordinary adaptability and amplified efficacy. These compounds exhibit heightened selectivity and specificity, significantly augmenting the overall effectiveness of PDT. The ongoing evolution spans three generational tiers, striving relentlessly for amplified efficacy and heightened tumor selectivity.
Transition metal coordination complexes have emerged as pivotal players in PDT. These complexes, exemplified by ruthenium (II) and iridium (III) complexes, extend excited state interactions with molecular oxygen, enhancing PDT efficacy by elevating light absorption at longer wavelengths and exhibiting substantial molar extinction coefficients. Their integration into photosensitizers amplifies therapeutic effectiveness and specificity.
The integration of cutting-edge nanomaterials, such as Metal-Organic Frameworks (MOFs) and organic fluorophore-based photosensitizers, has reshaped PDT paradigms. MOFs, characterized by high porosity and customizable pore dimensions, facilitate targeted photosensitizer delivery, significantly boosting efficacy. Organic fluorophore-based photosensitizers ensure low toxicity and remarkable biocompatibility, enhancing treatment precision and pharmacokinetics. These nanomaterials pave the way for meticulous targeting, controlled release, and improved treatment efficacy.
As summarised in Table 1 tabulation, recent strides in PDT present a spectrum of benefits, including enhanced light penetration in cancerous tissues, refined tumor targeting, and augmented therapy potency. Nonetheless, challenges persist, such as the complexity of synthesizing photosensitizers, their limited solubility, and concerns related to photostability and cancer cell selectivity. Thorough safety assessments are imperative before clinical translation to ensure sustained safety and therapeutic efficacy.
Table 1. Tabulation of the significance of recent advancements in PDT. |
|
Recent advancements |
Benefits |
Photosensitizer evolution |
A. Enhanced light penetration leading to increased efficacy in cancerous regions. B. Improved tumor selectivity through amalgamation or encapsulation methods. C. Augmented selectivity and specificity, especially in metal-complex-based pss
|
Metal complexes in ps |
A. Notable attributes such as structural adaptability and prolonged emissions duration. B. Significant interest among researchers due to favorable properties. C. Enhanced pdt efficacy, evidenced by increased light absorption and substantial molar extinction coefficient.
|
Nanomaterial integration |
A. High porosity, specific surface area, and modifiable pore dimensions in mofs. B. Enhanced photosensitizer accumulation within targeted cells during pdt. C. Low toxicity, commendable biocompatibility, and enduring triplet lifetimes in organic fluorophore-based pss. D. Improved safety and efficacy of pss through precise targeting, controlled release, and enhanced pharmacokinetics. |
None.
Availability of data and materials
Data and materials are available on request from the authors.
Ethical policy
Not applicable.
Author contributions
SDK conceptualized, designed, conducted research, and wrote the first draft. KJ provided supervision and revision of the draft.
Competing interests
The authors have no conflicts of interest regarding the publication of this paper.
Funding
None.
- Dolmans DE, Fukumura D, Jain RK: Photodynamic therapy for cancer. Nat Rev Cancer 2003, 3(5): 380-387.
- Lo KK-W, Louie M-W, Zhang KY: Design of luminescent iridium (III) and rhenium (I) polypyridine complexes as in vitro and in vivo ion, molecular and biological probes. Coord Chem Rev 2010, 254(21-22): 2603-2622.
- Castano AP, Demidova TN, Hamblin MR: Mechanisms in photodynamic therapy: part one-photosensitizers, photochemistry and cellular localization. Photodiagnosis Photodyn Ther 2004, 1(4): 279-293.
- Yanovsky RL, Bartenstein DW, Rogers GS, Isakoff SJ, Chen ST: Photodynamic therapy for solid tumors: A review of the literature. Photodermatol Photoimmunol Photomed 2019, 35(5): 295-303.
- Soliman N, Sol V, Ouk TS, Thomas CM, Gasser G: Encapsulation of a Ru(II) Polypyridyl Complex into Polylactide Nanoparticles for Antimicrobial Photodynamic Therapy. Pharmaceutics 2020, 12(10): 961.
- Yawalkar MM, Menon S, Swart HC, Dhoble SJ: Fundamentals of photodynamic therapy. In: Photophys Nanophys Ther. Epub ahead of print., edn.: Elsevier; 2022: 51-88.
- Villemin E, Ong YC, Thomas CM, Gasser G: Polymer encapsulation of ruthenium complexes for biological and medicinal applications. Nat Rev Chem 2019, 3(4): 261-282.
- Zhao X, Liu J, Fan J, Chao H, Peng X: Recent progress in photosensitizers for overcoming the challenges of photodynamic therapy: from molecular design to application. Chem Soc Rev 2021, 50(6): 4185-4219.
- Abrahamse H, Hamblin MR: New photosensitizers for photodynamic therapy. Biochem J 2016, 473(4): 347-364.
- Wei F, Chen Z, Shen XC, Ji L, Chao H: Recent progress in metal complexes functionalized nanomaterials for photodynamic therapy. Chem Commun (Camb) 2023, 59(46): 6956-6968.
- Myrzakhmetov B, Arnoux P, Mordon S, Acherar S, Tsoy I, Frochot C: Photophysical Properties of Protoporphyrin IX, Pyropheophorbide-a and Photofrin(®) in Different Conditions. Pharmaceuticals (Basel) 2021, 14(2): 138.
- Liang X, Chen M, Bhattarai P, Hameed S, Dai Z: Perfluorocarbon@Porphyrin Nanoparticles for Tumor Hypoxia Relief to Enhance Photodynamic Therapy against Liver Metastasis of Colon Cancer. ACS Nano 2020, 14(10): 13569-13583.
- Sibata CH, Colussi VC, Oleinick NL, Kinsella TJ: Photodynamic therapy in oncology. Expert Opin Pharmacother 2001, 2(6): 917-927.
- Zeng L, Gupta P, Chen Y, Wang E, Ji L, Chao H, Chen ZS: The development of anticancer ruthenium(ii) complexes: from single molecule compounds to nanomaterials. Chem Soc Rev 2017, 46(19): 5771-5804.
- Monro S, Colón KL, Yin H, Roque J, 3rd, Konda P, Gujar S, Thummel RP, Lilge L, Cameron CG, McFarland SA: Transition Metal Complexes and Photodynamic Therapy from a Tumor-Centered Approach: Challenges, Opportunities, and Highlights from the Development of TLD1433. Chem Rev 2019, 119(2): 797-828.
- Ma DL, He HZ, Leung KH, Chan DS, Leung CH: Bioactive luminescent transition-metal complexes for biomedical applications. Angew Chem Int Ed Engl 2013, 52(30): 7666-7682.
- Shen J, Rees TW, Ji L, Chao H: Recent advances in ruthenium (II) and iridium (III) complexes containing nanosystems for cancer treatment and bioimaging. Coord Chem Rev 2021, 443: 214016.
- Kamkaew A, Lim SH, Lee HB, Kiew LV, Chung LY, Burgess K: BODIPY dyes in photodynamic therapy. Chem Soc Rev 2013, 42(1): 77-88.
- Qiu K, Zhu H, Rees TW, Ji L, Zhang Q, Chao H: Recent advances in lysosome-targeting luminescent transition metal complexes. Coord Chem Rev 2019, 398: 113010.
- Mahajan NM, Saini A, Raut NA, Dhoble SJ: Photophysics and Nanophysics in Therapeutics Epub ahead of print.: Elsevier; 2022.
- Agostinis P, Berg K, Cengel KA, Foster TH, Girotti AW, Gollnick SO, Hahn SM, Hamblin MR, Juzeniene A, Kessel D, et al: Photodynamic therapy of cancer: an update. CA Cancer J Clin 2011, 61(4): 250-281.
- Castano AP, Demidova TN, Hamblin MR: Mechanisms in photodynamic therapy: part two-cellular signaling, cell metabolism and modes of cell death. Photodiagnosis Photodyn Ther 2005, 2(1): 1-23.
- Fitzgerald F: Photodynamic Therapy (PDT) Epub ahead of print.: Nova Science Publishers, Incorporated; 2017.
- Ye Y, Zhao Y, Sun Y, Cao J: Recent Progress of Metal-Organic Framework-Based Photodynamic Therapy for Cancer Treatment. Int J Nanomedicine 2022, 17: 2367-2395.
Asia-Pacific Journal of Oncology
print ISSN: 2708-7980, online ISSN: 2708-7999
Copyright © Asia Pac J Oncol. This work is licensed under a Creative Commons Attribution-NonCommercial-No Derivatives 4.0 International (CC BY-NC-ND 4.0) License.