Review | Open Access
Current perspectives on glioblastoma treatment synergies between therapies and delivery systems
Debanjan Das1
1Institute of Pharmacology, First Faculty of Medicine, Charles University and General University Hospital in Prague, Prague, Czech Republic.
Correspondence: Debanjan Das (Institute of Pharmacology, First Faculty of Medicine, Charles University and General University Hospital in Prague, Prague, Czech Republic; E-mail: debanjan.imagine@outlook.com).
Asia-Pacific Journal of Oncology 2025, 6: 1-8. https://doi.org/10.32948/ajo.2024.12.30
Received: 18 Nov 2024 | Accepted: 30 Dec 2024 | Published online: 05 Jan 2025
Key words glioblastoma multiforme, delivery systems, central nervous system, stem cell, blood brain barrier
Glioblastoma multiformes (GBMs) are the most aggressive variant of glioma and a common malignant primary cerebral neoplasm, exhibiting an annual occurrence rate of 3.19 cases per 100,000 individuals in the general populace [4]. Glioblastoma prognosis remains grim, with only 4–5% of patients achieving five-year survival [5]. Notably, short-term outcomes are marginally more favorable, as 26–33% of individuals with this malignancy survive for up to two years following diagnosis [6]. Traditionally, GBMs have been associated with the brain [7]. However, current research has expanded our understanding, revealing that these malignant tumors may manifest in the brainstem, cerebellar regions, and spinal cord [8]. An analysis of the primary glioma distribution revealed that 61% of these neoplasms manifest within the four principal lobes of the cerebrum. The frontal lobe exhibited the highest incidence (25 %), while the temporal lobe accounted for 20% of the cases. The parietal and occipital lobes demonstrate lower frequencies, with 13% and 3% occurrences, respectively [9].
Gliomas are classified by the World Health Organization (WHO) into grades I to IV based on histological criteria that determine malignancy. Lesions with minimal proliferative potential characterize grade I gliomas, which can be effectively treated through curative surgical interventions. Gliomas classified as grades II−IV demonstrate pronounced malignancy and invasive characteristics. GBM distinguishes itself as the tumor variant exhibiting the most invasive behavior, highest degree of malignancy, and least developed cellular differentiation among this group. The WHO has categorized GBM as a grade IV neoplastic entity, reflecting its severe nature [10, 11]. Moreover, glioblastoma has been stratified into four distinct subtypes: proneural, neural, classical, and mesenchymal. Notably, the proneural subtype has a higher incidence among younger patient populations [12, 13]. The most current findings and diverse conclusions regarding GBM treatments must be widely disseminated throughout the scientific, medical, and research communities. As indicated in Figure 1, this comprehensive review elucidates and explores a range of cutting-edge drug delivery systems and therapeutic modalities presently utilized in managing GBM.
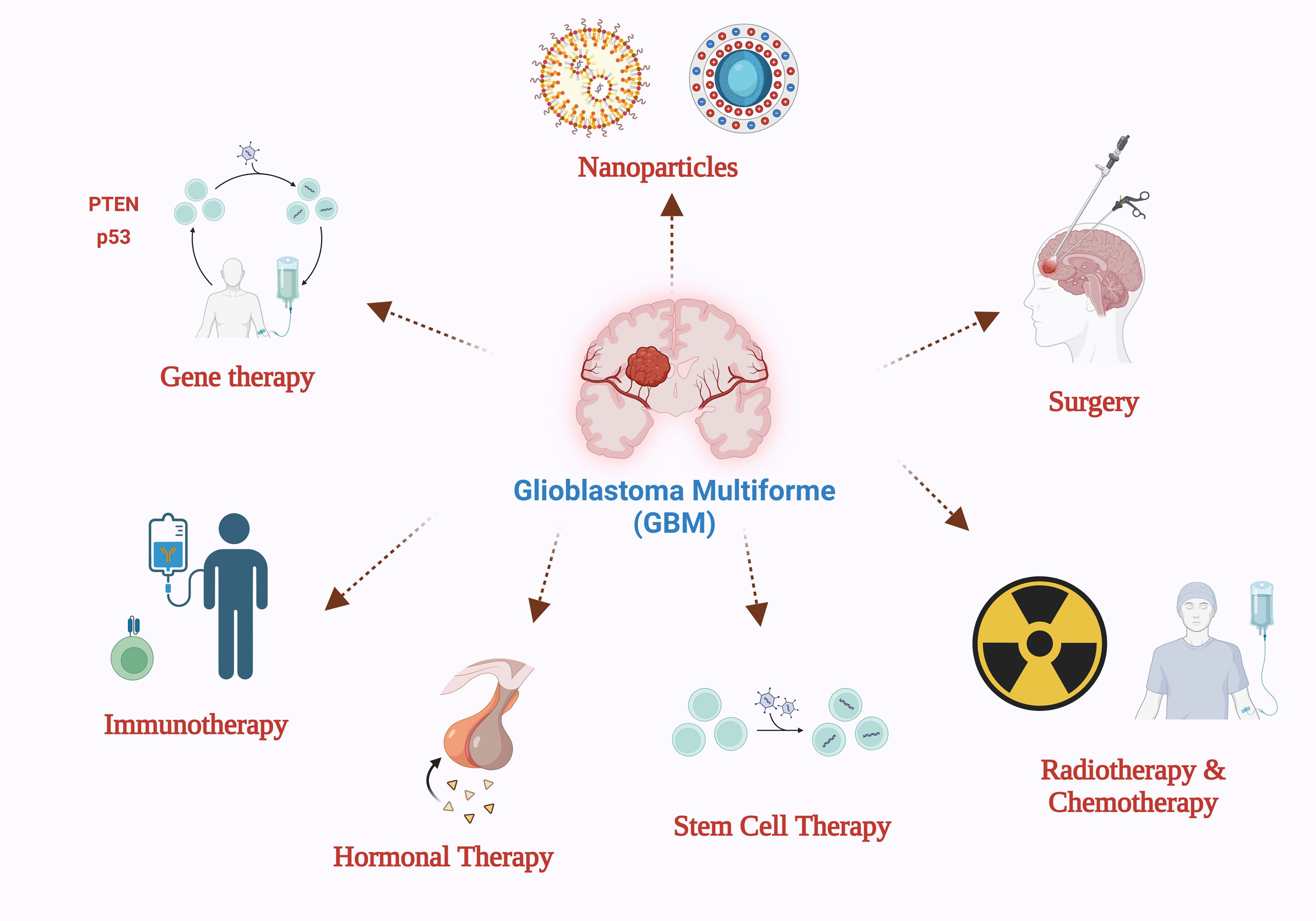
The majority of patients typically exhibit headaches, localized or progressive neurologic impairments, and elevated intracranial pressure as their symptoms. Seizures constitute the primary presenting symptom in 25% of patients, with potential recurrence observed in up to 50% of cases during later stages. Notably, approximately 13% of GBM instances may exhibit multifocal (exceeding two lesions), distant (secondary lesion non-contiguous with primary), or diffuse disease manifestations [26]. Advanced imaging techniques, specifically CT or MRI, are typically utilized in the initial diagnostic process for GBM. When using MRI, administering gadolinium contrast significantly enhances the visualization of most GBMs. These tumors characteristically manifest as irregularly contoured masses, exhibiting a dense enhancement ring surrounding a hypointense necrotic core [27]. The WHO grading system identifies necrosis as a critical component in diagnosing GBM. This distinctive feature is necessary for classifying a brain tumor as grade IV or GBM [28]. Additionally, diagnostic imaging may reveal the presence of peripheral vasogenic edema, hemorrhagic regions, and distortions in ventricular structure.
The neoplastic growth typically presents as an isolated, comparatively sizable, and irregularly contoured lesion that predominantly originates in the white matter. From a histological perspective, GBM shares characteristics with anaplastic astrocytoma, exhibiting a pleomorphic cellular composition that spans from small, poorly differentiated neoplastic elements to large, multinucleated cells. This presentation is accompanied by multifocal necrosis featuring pseudo-palisading nuclei and a high degree of mitotic activity [30, 31]. Vascular endothelial cell proliferation forming glomeruloid structures constitutes a significant defining feature.
GBM is classifiable into two forms: primary (de novo) and secondary. The origin of primary GBM is not linked to a known precursor, whereas secondary GBM evolves from the progression of a lower-grade glioma. The preponderance of GBM cases is classified as primary, with affected patients typically being older and demonstrating a less favorable clinical outcome in comparison to those diagnosed with secondary GBM [32]. The genetic pathogenesis of GBM follows two distinct routes. Primary glioblastoma results from the immediate conversion of glioma precursor cells, characteristically linking simultaneous modifications in multiple genes, notably EGFR and VEGFR. In contrast, secondary glioblastoma emerges through a gradual sequence of pathological alterations, with each stage linked to specific genetic modifications, including those affecting p53, CDK4, and P13K, among other genes [33].
During the late 1980s and early 1990s, the advent of frameless stereotaxy represented a significant turning point in brain tumor surgery. This innovative technique enabled surgeons to maneuver and place instruments precisely through image-guided systems. With the advancement of imaging technologies, frameless stereotaxy has evolved, enhancing the precision of surgical interventions for brain tumors [34]. Cerebral cortical stimulation has emerged as a crucial technique for intraoperative identification of eloquent brain regions that must be preserved during surgical procedures. This methodology, known as "brain mapping," enables surgeons to account for individual anatomical variations and potential tissue reorganization. By employing this approach, medical professionals can effectively excise tumors in areas of high functional significance while reducing the danger of compromising the patient's quality of life [35, 36].
The importance of imaging methodologies has grown substantially in GBM surgical procedures [37]. These imaging modalities serve to guide biopsy procedures, delineate tumor margins, and precisely localize critical brain structures that require preservation [38-40]. MRI emerges as the optimal technique for brain tumor imaging, offering unparalleled detail in anatomical structures and differentiation of soft tissues, which exceeds the capabilities of CT scanning and is useful for emergent imaging. Various MRI contrasts and pulse sequences can highlight critical tumor and brain tissue features, such as blood vessels, tumor necrosis, and hemorrhage (contrast-enhanced T1-weighted), cerebrospinal fluid (T2-weighted), and blood perfusion (dynamic susceptibility contrast (DSC), dynamic contrast-enhanced (DCE) and arterial spin labeling (ASL) [41, 42].
Intraoperative magnetic resonance imaging (iMRI) and intraoperative ultrasound (iUS) are valuable tools for neurosurgeons to detect residual malignant tissue during surgical interventions. Following the initial tumor excision, the surgeon conducts a contrast-enhanced MRI examination within the operating suite using specialized intraoperative imaging equipment. Should any residual enhancing tissue be observed, the surgeon proceeds to remove this additional tissue during the same operative session [43, 44].
Chemotherapy
The established post-operative therapeutic approach involves a 6-week course of simultaneous TMZ administration (75 mg/m²) and radiotherapy, succeeded by an adjuvant TMZ regimen (150–200 mg/m²) delivered for six cycles. The treatment was administered across five successive days, with each cycle separated by a 28-day interval [45]. Temozolomide (TMZ), a small-molecule alkylating agent, is the primary chemotherapeutic drug for GBM. This compound exerts its antitumor effects by directly inducing DNA damage through the methylation of purine bases [46, 47]. The formation of O6-methylguanine lesions constitutes the principal cytotoxic action, resulting in the initiation of apoptosis, autophagy, and cell degeneration. Furthermore, investigations have demonstrated that TMZ exhibits radiation-sensitizing effects. The concurrent administration of this agent with radiation therapy amplifies the potential for radiation-induced double-strand breaks in DNA, thereby increasing the likelihood of cellular demise [48, 49].
The exploration of nitrosourea-based compounds has been a significant avenue in GBM treatment research. One such agent, Carmustine, is characterized as a compact nitrogen mustard molecule that functions as an alkylating agent. Its action mechanism involves interstrand cross-links between guanine and cytosine nucleobases within the DNA structure [50, 51]. The therapeutic alkylating effects of Carmustine can be counteracted by the alkyl guanine transferase (AGT) enzyme. Consequently, Carmustine is often administered with AGT inhibitors to ensure optimal therapeutic efficacy. Lomustine, a member of the alkylating antitumor agent class, exhibits exceptional characteristics that set it apart from its counterparts. The compound's notable lipophilicity and compact molecular structure enable its efficient penetration of the blood brain barrier (BBB). These distinctive features contribute to lomustine's suitability for oral administration, enhancing its therapeutic potential in oncology [52].
The anti-angiogenic properties of bevacizumab, a humanized monoclonal antibody, are utilized through its intravenous administration. This therapeutic agent functions by connecting to and inhibiting vascular endothelial growth factor A (VEGF-A), a protein that, when bound to its receptor, triggers the development of fresh blood vessels [53, 54]. Bevacizumab exhibits a notable impact on progression-free survival in glioblastoma patients; however, it does not confer a substantial benefit in terms of overall survival for those newly diagnosed with this malignancy. Consequently, its primary utilization is focused on the treatment of recurrent glioblastoma cases [55].
Radiation therapy
Radiation therapy encompasses multiple modalities, such as x-ray photons, gamma photons, and protons; however, not all of these approaches have been substantiated as conventional therapeutic interventions. It can facilitate local control of the microscopic illness not addressed by surgical ablation. In CCRT, patients undergo 3D conforming radiotherapy, utilizing X-ray photons aimed at the tumor from many orientations. CT and MRI scans are employed to strategize administering the therapeutic dose to the tumor location. The standard protocol for radiation therapy involves the administration of 2 gray (Gy) fractions over a 6-week course, with cumulative doses ranging from 40 to 60 Gy [56]. In 80–90% of cases, recurrent gliomas typically emerge within a 2 cm radius of the initial tumor site, leading to the clinical adoption of conformal radiotherapy as the preferred treatment modality over whole-brain radiation [57]. Conformal radiotherapy is engineered to precisely target most residual GBM cells, simultaneously preserving the integrity of healthy cerebral tissue and minimizing cognitive adverse effects.
Stereotactic radiosurgery (SRS) represents an additional modality of focal radiation treatment, allowing for the application of significantly elevated radiation doses [58]. This is achieved through the utilization of multiple converging radiation beams that are non-parallel. The radiation protocol employed in SRS is characterized by administering higher doses per fraction, typically exceeding 15 Gy and a reduced number of fractions [59]. The method employs a cobalt-60 source to generate gamma radiation, accurately focused on the tumor using custom-designed beam shapers. SRS has gained widespread acceptance in treating brain metastasis, primarily due to its ability to reduce the cognitive complications associated with whole-brain radiotherapy. In certain instances of GBM, SRS might be considered an appropriate treatment approach [60].
NPs demonstrate the capacity to diminish the effectiveness of certain active pharmaceutical ingredients. These microscopic structures serve as protective agents, shielding medications from enzymatic and chemical degradation. Additionally, NPs can facilitate targeted delivery of various active compounds, including cytostatic agents, antitoxins, proteins, nucleic acids, and polypeptides, to specific tissues. Additionally, the versatility of NPs allows for their administration through diverse routes, encompassing oral, percutaneous, intra-ophthalmic, intranasal, and intravascular pathways [64].
Novel theranostic nanocarriers provide real-time imaging and treatment of diseases. The development of theranostic agents remains challenging. A multifunctional theranostic nano system based on dendrimers was developed for malignant tumor cell chemotherapy and CT imaging, emphasising specification [65]. The synthesis of a fifth-generation (G5) polyamidoamine (PAMAM) dendrimer incorporated doxorubicin (DOX), an exemplary antineoplastic agent, through an acid-labile cis-aconityl (NHAc) bond. The dendrimer was further modified by prefunctionalization with folic acid (FA) to yield the final G5 construct. Gold nanoparticles (Au NPs) were also employed to encapsulate NHAc−FA−DOX conjugated mixtures, forming Au NP dendrimers (Au DENPs). The DOX-conjugated Au DENPs demonstrates promising potential for simultaneous implementation in chemotherapeutic treatment and CT imaging across various classifications of malignant neoplasms [66].
The effectiveness of anticancer medications in GBM treatment is hampered by three primary factors: increased systemic toxicity, inadequate cancer cell-specific targeting, and suboptimal penetration of the BBB. In response to these constraints, researchers have turned to solid lipid nanoparticles (SLNPs) as a possible solution. These nanoparticles demonstrate enhanced biocompatibility and reduced systemic toxicity compared to conventional drug delivery approaches and other anticancer agents currently used for GBM treatment. The outermost portion of solid lipid nanoparticles was conjugated with lipoprotein receptor-related protein 1 (LRP1) and angiopep-2, a ligand found in the epithelial cells of both the brain and glioma, for the delivery of docetaxel (DTX). The Angiopep-2 linked solid lipid nanoparticles (A-SLN) demonstrated enhanced cytotoxicity, superior cellular uptake, and encouraging apoptosis relative to the blank (unconjugated SLNPs) against U87MG human glioblastoma and GL261 murine glioma cells. Furthermore, tissue distribution and pharmacokinetic evaluations demonstrated that A-SLN exhibited enhanced brain-specific targeting and accumulation compared to the commercially available DTX formulation (Taxotere). The findings from these studies suggest that A-SLN could be a valuable therapeutic approach for addressing GBM [67].
Surface characterization studies have revealed the potential of zinc oxide (ZnO) nanoparticles (NPs) as a therapeutic agent in cancer treatment. A particular investigation focused on the relationship between ZnO NPs and three predominant blood proteins: albumin, fibrinogen, and apo-transferrin. These proteins were attached to ZnO NPs through two distinct mechanisms: covalent bonding (c-ZnO NPs) and non-specific adsorption (n-ZnO NPs). The research sought to elucidate how these alternative attachment methods influenced protein structure and their subsequent effects on GBM cells. Fourier-transform infrared spectroscopy (FTIR) examined the protein structures and modification of ZnO NPs. FTIR study showed that noncovalent contact considerably impacted the secondary structure of proteins compared to those covalently bound to ZnO NPs. The experimental findings revealed that albumin-conjugated c-ZnO NPs and apo-transferrin exhibited a dual effect on U373 cells at subtoxic concentrations. These compounds interfered with cellular cycle progression while mitigating the necrotic cell death rate. Such observations suggest potential therapeutic implications for the treatment of GBM cells [68].
Celecoxib, a selective cyclo-oxygenase-2 (COX-2) inhibitor, demonstrates its therapeutic effects by modulating apoptotic pathways and cellular growth mechanisms [69]. Investigations have revealed that nanoparticles comprising celecoxib-conjugated poly(lactic-co-glycolic acid) (PLGA) exhibit substantial antineoplastic activity against glioma cell lines, specifically U87MG and C6, with effectiveness correlating to the administered dose. These findings suggest that celecoxib-functionalized PLGA nanoparticles constitute a promising drug delivery system for the management of GBM [70].
Emerging research has revealed that glioblastoma harbors a distinct subclass of cells recognized as glioma stem cells (GSCs). These GSCs exhibit remarkable characteristics, including self-renewal potential and heightened proliferative capacity, attributing to the swift onset of tumor infiltration and metastasis [72, 73]. The management of GSCs plays a pivotal role in averting tumor recurrence. Normal SCs migrate toward tumor sites and interact with CSCs, making them valuable tools for targeting GSCs in GB therapy. The interplay between normal SCs and GSCs suppresses tumor proliferation, angiogenesis, and metastasis while diminishing inflammation and apoptosis. Studies have demonstrated that human MSCs and HSCs can specifically target GSCs and impede GB progression [74, 75].
Antiproliferative proteins represent a category of biological agents that impede the association between native ligands and their corresponding receptors. A prime illustration of this mechanism is the obstruction of epidermal growth factor (EGF) attachment to its receptor variant III (EGFRvIII). These characteristics position these proteins as an ideal therapeutic avenue for GB, given their capacity to interfere with essential cellular communication pathways [76]. As a result, a decrease in the growth of cancerous cells was revealed [77, 78]. In antiproliferative agents, a select subset possesses the unique ability to be extruded into the extracellular space. Interferons α (IFN-α) and β (IFN-β) have been shown to influence tumor cell growth in diverse pre-clinical cancer models. A recent investigation evaluated the effects of IFN-β, a representative cytokine expressed by therapeutic SCs, on glioblastoma progression in murine models. The findings demonstrated that systemic administration of this cytokine reduced tumor growth [79].
Bone morphogenetic proteins (BMPs) can impede tumor progression by activating their corresponding receptors (BMPRs). Furthermore, BMP4 secreted by human adipose-derived mesenchymal stem cells demonstrates efficacy in suppressing tumor growth and enhancing the longevity of mice afflicted with glioblastoma [80, 81]. Certain SC proteins, notably growth arrest specific-1 (Gas1), trigger cell cycle arrest and demonstrate antitumoral properties against glioblastoma [82]. SC therapy is emerging as a potentially transformative approach to treating glioblastoma. As extensively discussed, SCs possess an innate ability to home in on tumor sites, making them mainly ideal for the delivery of anticancer agents. To address the challenges of traditional drug delivery systems (DDSs), often hampered by brief half-lives, both SCs and their secreted extracellular vesicles (EVs) can express or encapsulate various antitumor payloads. Moreover, SCs demonstrate potential in detecting brain tumor masses, enhancing their value in glioblastoma management.
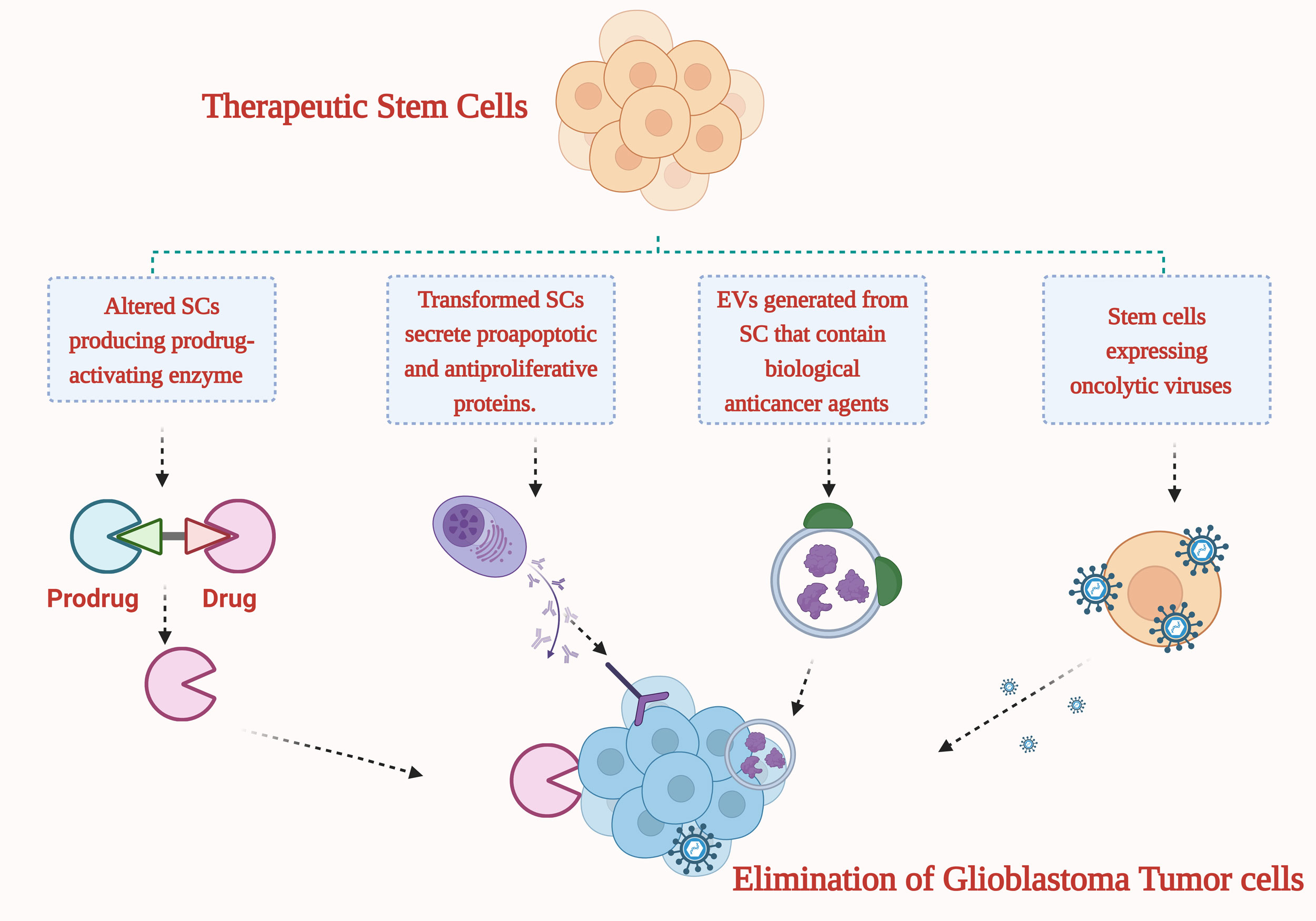
No applicable.
Ethics approval
No applicable.
Data availability
The data will be available upon request.
Funding
None.
Authors’ contribution
DD contributed to the conception, design, writing of this review article, figures drawing and submitted the final version of the manuscript.
Competing interests
None.
- Holland EC: Glioblastoma multiforme: the terminator. Proc Natl Acad Sci U S A 2000, 97(12): 6242-6244.
- Agnihotri S, Burrell KE, Wolf A, Jalali S, Hawkins C, Rutka JT, Zadeh G: Glioblastoma, a brief review of history, molecular genetics, animal models and novel therapeutic strategies. Arch Immunol Ther Exp (Warsz) 2013, 61(1): 25-41.
- Messali A, Villacorta R, Hay JW: A review of the economic burden of glioblastoma and the cost effectiveness of pharmacologic treatments. Pharmacoeconomics 2014, 32(12): 1201-1212.
- Zheng X, Tang Q, Ren L, Liu J, Li W, Fu W, Wang J, Du G: A narrative review of research progress on drug therapies for glioblastoma multiforme. Ann Transl Med 2021, 9(11): 943.
- Hanif F, Muzaffar K, Perveen K, Malhi SM, Simjee SU: Glioblastoma multiforme: a review of its epidemiology and pathogenesis through clinical presentation and treatment. Asian Pac J Cancer Prev 2017, 18(1): 3-9.
- Davis ME: Epidemiology and overview of gliomas. Semin Oncol Nurs 2018, 34(5): 420-429.
- Yalamarty SSK, Filipczak N, Li X, Subhan MA, Parveen F, Ataide JA, Rajmalani BA, Torchilin VP: Mechanisms of resistance and current treatment options for glioblastoma multiforme (GBM). Cancers 2023, 15(7): 2116.
- Ghosh MK, Kumar S, Begam S, Ghosh S, Basu M: GBM immunotherapy: Exploring molecular and clinical frontiers. Life Sci 2024 1(356): 123018
- Lovely MP, Stewart-Amidei C, Arzbaecher J, Bell S, Maher ME, Maida M, Mogensen K, Nicolaseau G: Care of the adult patient with a brain tumor. J Neurosci Nurs 2014, 46(6): 367-369.
- Louis DN, Ohgaki H, Wiestler OD, Cavenee WK, Burger PC, Jouvet A, Scheithauer BW, Kleihues P: The 2007 WHO classification of tumours of the central nervous system. Acta Neuropathol 2007, 114(2): 97-109.
- Jovčevska I, Kočevar N, Komel R: Glioma and glioblastoma - how much do we (not) know? Mol Clin Oncol 2013, 1(6): 935-941.
- Oronsky B, Reid TR, Oronsky A, Sandhu N, Knox SJ: A Review of Newly Diagnosed Glioblastoma. Front Oncol 2020, 10: 574012.
- Taylor OG, Brzozowski JS, Skelding KA: Glioblastoma Multiforme: An Overview of Emerging Therapeutic Targets. Front Oncol 2019, 9: 963.
- Iacob G, Dinca EB: Current data and strategy in glioblastoma multiforme. J Med Life 2009, 2(4): 386-393.
- Ohgaki H, Kleihues P: The definition of primary and secondary glioblastoma. Clin Cancer Res 2013, 19(4): 764-772.
- Barnholtz-Sloan JS, Ostrom QT, Cote D: Epidemiology of Brain Tumors. Neurol Clin 2018, 36(3): 395-419.
- Fisher JL, Schwartzbaum JA, Wrensch M, Wiemels JL: Epidemiology of brain tumors. Neurol Clin 2007, 25(4): 867-890, vii.
- Simińska D, Korbecki J, Kojder K, Kapczuk P, Fabiańska M, Gutowska I, Machoy-Mokrzyńska A, Chlubek D, Baranowska-Bosiacka I: Epidemiology of Anthropometric Factors in Glioblastoma Multiforme-Literature Review. Brain Sci 2021, 11(1): 116.
- Ohgaki H: Epidemiology of brain tumors. Methods Mol Biol 2009, 472: 323-342.
- Hanif F, Muzaffar K, Perveen K, Malhi SM, Simjee Sh U: Glioblastoma Multiforme: A Review of its Epidemiology and Pathogenesis through Clinical Presentation and Treatment. Asian Pac J Cancer Prev 2017, 18(1): 3-9.
- Johnson DR, Fogh SE, Giannini C, Kaufmann TJ, Raghunathan A, Theodosopoulos PV, Clarke JL: Case-Based Review: newly diagnosed glioblastoma. Neurooncol Pract 2015, 2(3): 106-121.
- Kuang H, Jiang N, Jia XY, Cui Z, Zhao MH: Epidemiology, clinical features, risk factors, and outcomes in anti-glomerular basement membrane disease: A systematic review and meta-analysis. Autoimmun Rev 2024, 23(4): 103531.
- Alifieris C, Trafalis DT: Glioblastoma multiforme: Pathogenesis and treatment. Pharmacol Ther 2015, 152: 63-82.
- Alexander BM, Cloughesy TF: Adult Glioblastoma. J Clin Oncol 2017, 35(21): 2402-2409.
- Young RM, Jamshidi A, Davis G, Sherman JH: Current trends in the surgical management and treatment of adult glioblastoma. Ann Transl Med 2015, 3(9): 121.
- Schiff D, Lee EQ, Nayak L, Norden AD, Reardon DA, Wen PY: Medical management of brain tumors and the sequelae of treatment. Neuro Oncol 2015, 17(4): 488-504.
- Feyissa AM, Sanchez-Boluarte SS, Moniz-Garcia D, Chaichana KL, Sherman WJ, Freund BE, Tatum WO, Middlebrooks EH, Sirven JI, Quinones-Hinojosa A: Risk factors for preoperative and postoperative seizures in patients with glioblastoma according to the 2021 World Health Organization classification. Seizure 2023, 112: 26-31.
- Thakur A, Faujdar C, Sharma R, Sharma S, Malik B, Nepali K, Liou JP: Glioblastoma: Current Status, Emerging Targets, and Recent Advances. J Med Chem 2022, 65(13): 8596-8685.
- Nakada M, Kita D, Watanabe T, Hayashi Y, Teng L, Pyko IV, Hamada J: Aberrant signaling pathways in glioma. Cancers (Basel) 2011, 3(3): 3242-3278.
- Agnihotri S, Burrell KE, Wolf A, Jalali S, Hawkins C, Rutka JT, Zadeh G: Glioblastoma, a brief review of history, molecular genetics, animal models and novel therapeutic strategies. Arch Immunol Ther Exp (Warsz) 2013, 61(1): 25-41.
- Frosch M: Central nervous system in Robbins basic pathology. Kumar V, Abbas AK, Astor JC. In. Epub ahead of print.: Elsevier Saunders, Philadelphia; 2013.
- Khabibov M, Garifullin A, Boumber Y, Khaddour K, Fernandez M, Khamitov F, Khalikova L, Kuznetsova N, Kit O, Kharin L: Signaling pathways and therapeutic approaches in glioblastoma multiforme (Review). Int J Oncol 2022, 60(6): 69.
- Behrooz AB, Latifi-Navid H, Nezhadi A, Świat M, Los M, Jamalpoor Z, Ghavami S: Molecular mechanisms of microRNAs in glioblastoma pathogenesis. Biochim Biophys Acta Mol Cell Res 2023, 1870(6): 119482.
- Azagury DE, Dua MM, Barrese JC, Henderson JM, Buchs NC, Ris F, Cloyd JM, Martinie JB, Razzaque S, Nicolau S, et al: Image-guided surgery. Curr Probl Surg 2015, 52(12): 476-520.
- Watts C, Sanai N: Surgical approaches for the gliomas. Handb Clin Neurol 2016, 134: 51-69.
- Ojemann JG, Miller JW, Silbergeld DL: Preserved function in brain invaded by tumor. Neurosurgery 1996, 39(2): 253-258; discussion 258-259.
- Moore CM, Robertson NL, Arsanious N, Middleton T, Villers A, Klotz L, Taneja SS, Emberton M: Image-guided prostate biopsy using magnetic resonance imaging-derived targets: a systematic review. Eur Urol 2013, 63(1): 125-140.
- Kobayashi K, Bhargava P, Raja S, Nasseri F, Al-Balas HA, Smith DD, George SP, Vij MS: Image-guided biopsy: what the interventional radiologist needs to know about PET/CT. Radiographics 2012, 32(5): 1483-1501.
- Klimberg VS, Rivere A: Ultrasound image-guided core biopsy of the breast. Chin Clin Oncol 2016, 5(3): 33.
- Hu S, Kang H, Baek Y, El Fakhri G, Kuang A, Choi HS: Real-Time Imaging of Brain Tumor for Image-Guided Surgery. Adv Healthc Mater 2018, 7(16): e1800066.
- Villanueva-Meyer JE, Mabray MC, Cha S: Current Clinical Brain Tumor Imaging. Neurosurgery 2017, 81(3): 397-415.
- Pan JW, Moon CH, Hetherington HP: Cerebrospinal fluid-suppressed T(2) -weighted MR imaging at 7 T for human brain. Magn Reson Med 2019, 81(5): 2924-2936.
- Nimsky C, Ganslandt O, Hastreiter P, Fahlbusch R: Intraoperative compensation for brain shift. Surg Neurol 2001, 56(6): 357-364.
- Ohue S, Kumon Y, Nagato S, Kohno S, Harada H, Nakagawa K, Kikuchi K, Miki H, Ohnishi T: Evaluation of intraoperative brain shift using an ultrasound-linked navigation system for brain tumor surgery. Neurol Med Chir (Tokyo) 2010, 50(4): 291-300.
- Stupp R, Mason WP, van den Bent MJ, Weller M, Fisher B, Taphoorn MJ, Belanger K, Brandes AA, Marosi C, Bogdahn U, et al: Radiotherapy plus concomitant and adjuvant temozolomide for glioblastoma. N Engl J Med 2005, 352(10): 987-996.
- Syro LV, Rotondo F, Camargo M, Ortiz LD, Serna CA, Kovacs K: Temozolomide and Pituitary Tumors: Current Understanding, Unresolved Issues, and Future Directions. Front Endocrinol (Lausanne) 2018, 9: 318.
- Knizhnik AV, Roos WP, Nikolova T, Quiros S, Tomaszowski KH, Christmann M, Kaina B: Survival and death strategies in glioma cells: autophagy, senescence and apoptosis triggered by a single type of temozolomide-induced DNA damage. PLoS One 2013, 8(1): e55665.
- Meikrantz W, Bergom MA, Memisoglu A, Samson L: O6-alkylguanine DNA lesions trigger apoptosis. Carcinogenesis 1998, 19(2): 369-372.
- Chakravarti A, Erkkinen MG, Nestler U, Stupp R, Mehta M, Aldape K, Gilbert MR, Black PM, Loeffler JS: Temozolomide-mediated radiation enhancement in glioblastoma: a report on underlying mechanisms. Clin Cancer Res 2006, 12(15): 4738-4746.
- Brent TP, Remack JS: Formation of covalent complexes between human O6-alkylguanine-DNA alkyltransferase and BCNU-treated defined length synthetic oligodeoxynucleotides. Nucleic Acids Res 1988, 16(14b): 6779-6788.
- Grossman SA, Reinhard C, Colvin OM, Chasin M, Brundrett R, Tamargo RJ, Brem H: The intracerebral distribution of BCNU delivered by surgically implanted biodegradable polymers. J Neurosurg 1992, 76(4): 640-647.
- Bartzatt R: Lomustine analogous drug structures for intervention of brain and spinal cord tumors: the benefit of in silico substructure search and analysis. Chemother Res Pract 2013, 2013: 360624.
- Friedman HS, Prados MD, Wen PY, Mikkelsen T, Schiff D, Abrey LE, Yung WK, Paleologos N, Nicholas MK, Jensen R, et al: Bevacizumab alone and in combination with irinotecan in recurrent glioblastoma. J Clin Oncol 2009, 27(28): 4733-4740.
- Ozel O, Kurt M, Ozdemir O, Bayram J, Akdeniz H, Koca D: Complete response to bevacizumab plus irinotecan in patients with rapidly progressive GBM: Cases report and literature review. J Oncol Sci 2016, 2(2-3): 87-94.
- Diaz RJ, Ali S, Qadir MG, De La Fuente MI, Ivan ME, Komotar RJ: The role of bevacizumab in the treatment of glioblastoma. J Neurooncol 2017, 133(3): 455-467.
- Cabrera AR, Kirkpatrick JP, Fiveash JB, Shih HA, Koay EJ, Lutz S, Petit J, Chao ST, Brown PD, Vogelbaum M, et al: Radiation therapy for glioblastoma: Executive summary of an American Society for Radiation Oncology Evidence-Based Clinical Practice Guideline. Pract Radiat Oncol 2016, 6(4): 217-225.
- Hochberg FH, Pruitt A: Assumptions in the radiotherapy of glioblastoma. Neurology 1980, 30(9): 907-911.
- Ganz JC: The journey from proton to gamma knife. Prog Brain Res 2014, 215: 67-75.
- Leber KA, Berglöff J, Pendl G: Dose-response tolerance of the visual pathways and cranial nerves of the cavernous sinus to stereotactic radiosurgery. J Neurosurg 1998, 88(1): 43-50.
- Redmond KJ, Mehta M: Stereotactic Radiosurgery for Glioblastoma. Cureus 2015, 7(12): e413.
- Li B, Shao H, Gao L, Li H, Sheng H, Zhu L: Nano-drug co-delivery system of natural active ingredients and chemotherapy drugs for cancer treatment: a review. Drug Deliv 2022, 29(1): 2130-2161.
- De Jong WH, Borm PJ: Drug delivery and nanoparticles:applications and hazards. Int J Nanomedicine 2008, 3(2): 133-149.
- Madkhali OA: Perspectives and Prospective on Solid Lipid Nanoparticles as Drug Delivery Systems. Molecules 2022, 27(5): 1543.
- Tzeng SY, Green JJ: Therapeutic nanomedicine for brain cancer. Ther Deliv 2013, 4(6): 687-704.
- Zhu J, Wang G, Alves CS, Tomás H, Xiong Z, Shen M, Rodrigues J, Shi X: Multifunctional Dendrimer-Entrapped Gold Nanoparticles Conjugated with Doxorubicin for pH-Responsive Drug Delivery and Targeted Computed Tomography Imaging. Langmuir 2018, 34(41): 12428-12435.
- Hu D, Xu H, Xiao B, Li D, Zhou Z, Liu X, Tang J, Shen Y: Albumin-Stabilized Metal-Organic Nanoparticles for Effective Delivery of Metal Complex Anticancer Drugs. ACS Appl Mater Interfaces 2018, 10(41): 34974-34982.
- Kadari A, Pooja D, Gora RH, Gudem S, Kolapalli VRM, Kulhari H, Sistla R: Design of multifunctional peptide collaborated and docetaxel loaded lipid nanoparticles for antiglioma therapy. Eur J Pharm Biopharm 2018, 132: 168-179.
- Altunbek M, Keleştemur S, Baran G, Çulha M: Role of modification route for zinc oxide nanoparticles on protein structure and their effects on glioblastoma cells. Int J Biol Macromol 2018, 118(Pt A): 271-278.
- Tsujii M, Kawano S, Tsuji S, Sawaoka H, Hori M, DuBois RN: Cyclooxygenase regulates angiogenesis induced by colon cancer cells. Cell 1998, 93(5): 705-716.
- Suzuki K, Gerelchuluun A, Hong Z, Sun L, Zenkoh J, Moritake T, Tsuboi K: Celecoxib enhances radiosensitivity of hypoxic glioblastoma cells through endoplasmic reticulum stress. Neuro Oncol 2013, 15(9): 1186-1199.
- Guardia GDA, Correa BR, Araujo PR, Qiao M, Burns S, Penalva LOF, Galante PAF: Proneural and mesenchymal glioma stem cells display major differences in splicing and lncRNA profiles. NPJ Genom Med 2020, 5: 2.
- Bao S, Wu Q, McLendon RE, Hao Y, Shi Q, Hjelmeland AB, Dewhirst MW, Bigner DD, Rich JN: Glioma stem cells promote radioresistance by preferential activation of the DNA damage response. Nature 2006, 444(7120): 756-760.
- Schulz A, Meyer F, Dubrovska A, Borgmann K: Cancer Stem Cells and Radioresistance: DNA Repair and Beyond. Cancers (Basel) 2019, 11(6): 862.
- Pacioni S, D'Alessandris QG, Giannetti S, Morgante L, Coccè V, Bonomi A, Buccarelli M, Pascucci L, Alessandri G, Pessina A, et al: Human mesenchymal stromal cells inhibit tumor growth in orthotopic glioblastoma xenografts. Stem Cell Res Ther 2017, 8(1): 53.
- Bryukhovetskiy IS, Dyuizen IV, Shevchenko VE, Bryukhovetskiy AS, Mischenko PV, Milkina EV, Khotimchenko YS: Hematopoietic stem cells as a tool for the treatment of glioblastoma multiforme. Mol Med Rep 2016, 14(5): 4511-4520.
- Rutkowska A, Stoczyńska-Fidelus E, Janik K, Włodarczyk A, Rieske P: EGFR(vIII): An Oncogene with Ambiguous Role. J Oncol 2019, 2019: 1092587.
- van de Water JA, Bagci-Onder T, Agarwal AS, Wakimoto H, Roovers RC, Zhu Y, Kasmieh R, Bhere D, Van Bergen en Henegouwen PM, Shah K: Therapeutic stem cells expressing variants of EGFR-specific nanobodies have antitumor effects. Proc Natl Acad Sci U S A 2012, 109(41): 16642-16647.
- Balyasnikova IV, Ferguson SD, Sengupta S, Han Y, Lesniak MS: Mesenchymal stem cells modified with a single-chain antibody against EGFRvIII successfully inhibit the growth of human xenograft malignant glioma. PLoS One 2010, 5(3): e9750.
- Nakamizo A, Marini F, Amano T, Khan A, Studeny M, Gumin J, Chen J, Hentschel S, Vecil G, Dembinski J, et al: Human bone marrow-derived mesenchymal stem cells in the treatment of gliomas. Cancer Res 2005, 65(8): 3307-3318.
- Ganaha S, Al-Kharboosh R, Ruiz-Valls A, Guerrero-Cazares H, Quinones-Hinojosa A: 216 human fat-derived mesenchymal stem cells bioengineered to secrete BMP4 are nononcogenic, suppress glioma, and prolong survival. Neurosurgery 2016, 63: 184.
- Mangraviti A, Tzeng SY, Gullotti D, Kozielski KL, Kim JE, Seng M, Abbadi S, Schiapparelli P, Sarabia-Estrada R, Vescovi A, et al: Non-virally engineered human adipose mesenchymal stem cells produce BMP4, target brain tumors, and extend survival. Biomaterials 2016, 100: 53-66.
- López-Ornelas A, Vergara P, Segovia J: Neural stem cells producing an inducible and soluble form of Gas1 target and inhibit intracranial glioma growth. Cytotherapy 2014, 16(7): 1011-1023.
Asia-Pacific Journal of Oncology
print ISSN: 2708-7980, online ISSN: 2708-7999
Copyright © Asia Pac J Oncol. This work is licensed under a Creative Commons Attribution-NonCommercial-No Derivatives 4.0 International (CC BY-NC-ND 4.0) License.